The treatment of PFAS-impacted groundwater using novel regenerable ion exchange resin: A five-year case study
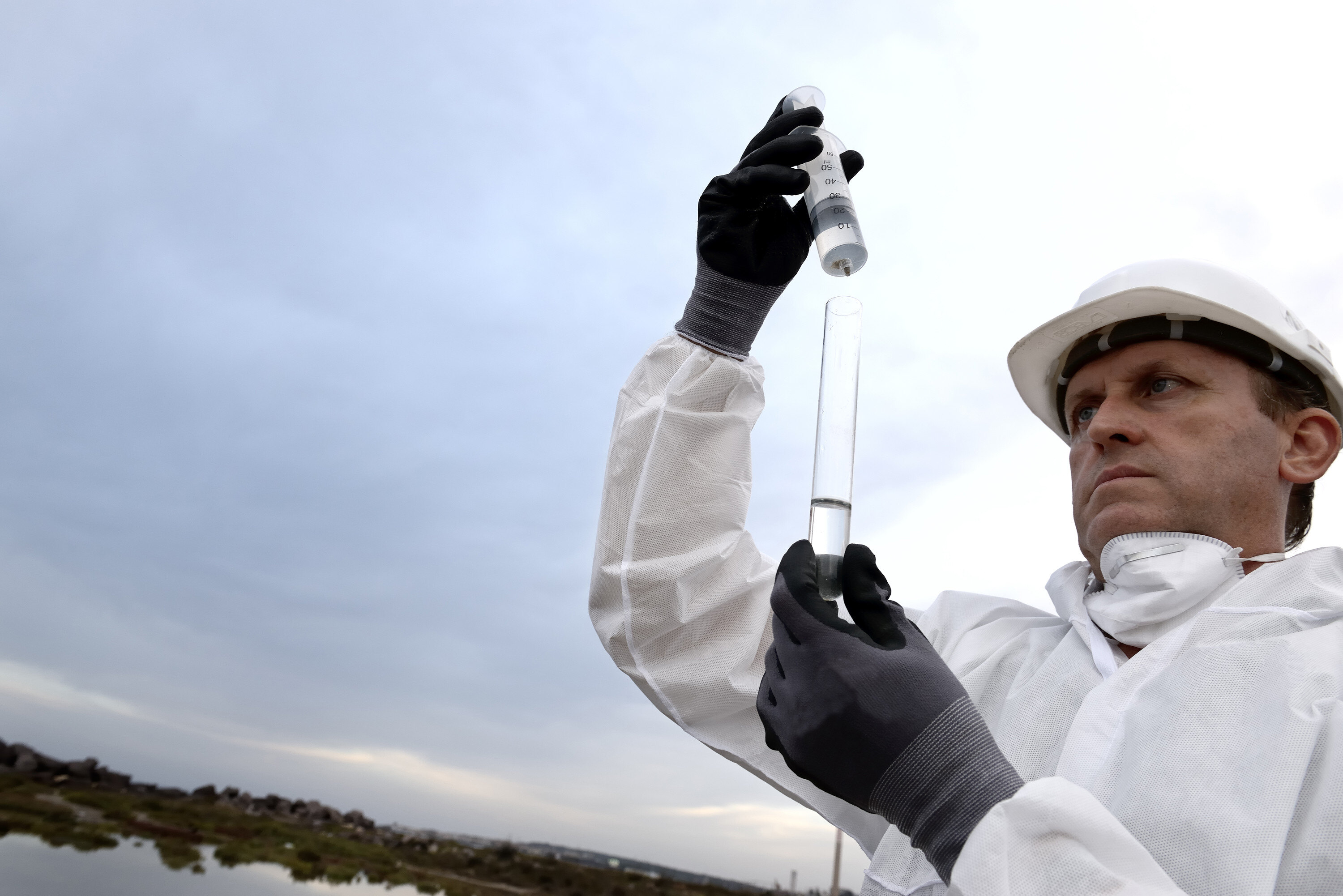
DOWNLOAD THE PAPER
Abstract
Regenerable ion exchange resin for PFAS remediation has been in service in Australia since September of 2017. Currently five regenerable systems are continuously operated for remediation purposes and another regenerable system is providing municipal drinking water treatment. This paper will provide an update on PFAS treatment using regenerable resin data from bench-, pilot-, and full-scale systems. The paper serves as a state-of-the-science for regenerable resin used in PFAS mitigation operations. Lessons learned from more than 5 years of full-scale operation are shared. A comprehensive break-down of the total water treated and mass of PFAS removed to date is provided. Resin optimisation work and data on the regeneration efficiency is discussed. Since 2019, 26 regeneration cycles have been performed at the example site, without build-up of residual PFAS on the resin. Additionally, no physical breakdown of the resin bead has been observed. From the regeneration perspective, a balance is performed on “mass PFAS removed” and the “mass PFAS recovered” during regeneration events.
Data is shared supporting these observations. Finally, economics of system performance are included.
Introduction
Per- and polyfluoroalkyl substances (PFAS) are contaminants that have increased in attention considerably in the last 10 years. Seemingly ubiquitous in the environment, PFAS have been found in a wide range of locations and applications. Examples of remote locations include both Antarctica and Tibet (Cousins et al., 2022). Additional evidence of PFAS pervasiveness includes identification in human blood and breastmilk, and in diverse products such as dental floss, ski wax, clothing, firefighting foams, microwave popcorn, cosmetics and in the electronics industry (Szilagyi, et.al., 2020; CDC, 2018; Zheng et al., 2021; Boronow et al., 2019; EPA, 2022a; Schellenberger et al., 2022, Backe et al., 2013, Seltenrich, 2020; Whitehead et al., 2021; Lay et.al., 2023). In fact, PFAS have been identified in almost all industrial branches. Glüge, et al. (2020) identified more than 200 use categories involving PFAS including, among others: the aerospace, biotechnology, chemical, electroplating, energy, oil and gas, and pharmaceutical sectors. It has been reported that rainwater often contains PFAS concentrations greater than various health advisories (Cousins et.al., 2022). Summarised, PFAS are in the air that we breathe (Morales-McDevitt, et. al., 2021), the water that we drink (Crone, et al., 2019), and the soil that grows our food (Felizeter, et. al., 2021). Presumptive contamination supported by retrospective site activities and modeling has suggested more than 57,000 sites in the United States are impacted with PFAS contamination (Salvatore, 2022). Much has been published about the adverse effects of PFAS to human health and the environment (Sunderland et.al., 2018; EPA, 2022b; EPA, 2022c; AU DOH, 2018, Cousins, 2022). Australian Drinking Water Guidelines have been established to protect human health and limit the combined total of PFOS and PFHxS to <0.070 μg/L and PFOA to <0.560 μg/L (AGI, 2024). When PFAS-impacted waters are identified and require remedy, various technologies have been implemented. Chief among full-scale treatment technologies are adsorbents such as granular activated carbon (GAC) and ion exchange resin (IX). Specific use-cases, site conditions, and customer preferences will dictate the choice of media but studies have found IX to be more cost effective with a lower environmental life cycle burden for the removal of both short and long chain PFAS when compared to GAC (Murray, 2021, Gagliano, 2020, Emery, 2018). The media (GAC or IX) eventually becomes exhausted of contaminant capacity and ultimate end-of-life considerations must be accounted for. Landfill disposal and thermal treatment are two possible actions. Future liability and tightening regulations are a concern for these options. The US EPA and others have suggested more study is needed to fully understand conditions required for complete decomposition and defluorination of PFAS through incineration (EPA 2024; EPA 2020; Baghirzade, 2021). Using a regenerable ion exchange (RIEX) resin is one approach minimising the generation of spent-media waste.
Ion exchange media is well established and proven for the removal of environmental contaminants (Bolto, 2002, Sen Gupta, 2017, Levchuk, 2017) including PFAS (Dixit, 2020; Gagliano, 2020, Fang, 2022). IX resins rely on two primary mechanisms for removal of contaminants: hydrophobic and electrostatic interactions. Due to the low pKa for the majority of anionic PFAS of concern, strong base anionic exchange resins are particularly well suited for PFAS remediation (Fang, 2022, Parker, 2022). Boyer (2022) identified PFAS removal efficacy as a function of the resin’s base polymer composition, functional group, and strong- or weak-base designation. Gagliano reported similar resin characteristics of importance and included resin porosity as another important factor influencing resin performance (2020). Liu (2021) found the PFAS properties of chain length, charge and functional group affected resin affinity at shorter contact times but beyond 2 hours, affinity was affected to a greater extent by the resin polymer matrix. Polystyrenic media achieved a higher removal efficiency for 40 legacy and emerging PFAS than polymethacrylate and polyacrylic resins (Liu, 2021). Dixit (2021) found similar results to Liu during his review.
Regenerable ion exchange media is also proven to be an effective treatment method for a variety of environmental contaminants (Sen Gupta, 2017) including PFAS (Woodard, 2018, Boyer, 2021, Feng, 2022). RIEX resins have been employed at full-scale at multiple locations starting in the mid- to late 2010s (Woodard, 2017; Woodard, 2018). In addition to the resin’s capacity for PFAS uptake, the regeneration efficiency must be sufficiently high to make regeneration viable. Both hydrophobic and electrostatic interactions must be overcome for effective regeneration. This is accomplished using a solvent (e.g., methanol, ethanol, isopropyl alcohol, or a mixture of these alcohols) to overcome the hydrophobic interactions (van der Wall forces or London dispersion forces), and a concentrated brine (e.g., NaCl) to reverse the resin’s preference for the ionically bound PFAS molecule. Multiple bed volumes of this solvent/brine solution are passed through the exhausted media bed to remove PFAS from the resin. Additionally, once removed from the resin, the PFAS must be able to diffuse back into the bulk solution. Gel resins, with less open pore structures often struggle kinetically in this regard. Liu (2021) found macroporus resins better suited for regeneration than gel-based resins and suggested size exclusion effects responsible for poor diffusion of PFAS from the gel resin. Regeneration is accomplished by passing several bed volumes of briny solvent through a bed of spent media removing PFAS from the resin and creating a concentrated solvent/salt/PFAS solution for further treatment. Liu (2021) tested 5 different salts and found NaCl and NH4Cl were the best performing brine ingredients for regeneration. Better results were seen with increasing brine concentrations but concentrations beyond 10% were not investigated (Liu, 2021). Studies have suggested that there is a limit to the brine concentration because of the decreasing PFAS solubility in higher ionic strength waters (Deng, 2016). Boyer (2021) investigated the environmental effects associated with 8 salts other than NaCl and found all alternative salts resulted in higher environmental impacts.
While research has been presented on the use of RIEX for PFAS removal, data and optimisation efforts from full-scale operations has not been widely shared. The objective of this effort is to share insights gained from a full-scale RIEX system in operation since March of 2019.
Method
Water quality
Initial remedial alternative investigations considered GAC, single-use ion exchange resin, and regenerable ion exchange resin for treatment of PFAS-impacted groundwater at the site. Concentrations in the groundwater before design commenced were reported to range from 16-320 μg/L for the sum of PFOS and PFHxS. Observed PFAS concentrations and other water quality parameters at the combined influent to the WTP are shown in Table 1. Water quality parameters are important to understand as excessive organics or monovalent or divalent ions can negatively affect resin capacity for the target of concern, PFAS. Treatment objectives were consistent with the Australian Drinking Water Guidance, with requirements of <0.560 μg/L for PFOA and <0.070 μg/L for the sum of PFOS + PFHxS. After considering the expected change-out frequencies and life-cycle costs of the different technologies, RIEX was selected as the treatment technology for full-scale implementation.
Table 1: Water quality characteristics
Site description
The treatment system consists of two stages of pre-treatment media operating in series, followed by two separate trains of lead-lag RIEX vessels operating in parallel. Following the two trains of RIEX, the streams are re-combined into a single line, which is passed through a single large polishing IEX stage before direct aquifer re-injection. Together, both trains process 12.6 L/sec of groundwater. A summary of the treatment system components is provided in Table 2.
Table 2: Treatment system components
Sample collection and analytical methods
All samples were collected in commercial laboratory supplied 20 ml HDPE bottles, stored at less than 6°C, and processed within a maximum of five days of sample collection. Concentrations of PFAS were determined at the subcontracted laboratory using LC/MS/MS and following a modified USEPA method 537.1, for which the lab is NATA accredited.
Standard methods for the analysis of water were followed for the determination of general water quality parameters reported in Table 1, all of which employed the use of NATA-accredited sub-contracted commercial labs.
Physical IEX analysis
Polystyrenic RIEX resin beads were submitted for physical analysis to compare the condition of resin beads repeatedly exposed to regeneration conditions against the condition of virgin RIEX beads. Samples of virgin, and regenerated resin beads were examined via microscopy and infrared spectroscopy. During this analysis, three drops of water were applied to an IR reflective slide, and multiple beads (~50-70) from each sample were placed into drops for visual inspection using a high-magnification camera (10x objective) within the laser direct infrared imaging (LDIR) system. The camera used a 10x objective imaging a 600 x 400 μm. Individual beads were crushed to facilitate infrared spectroscopy analysis using the LDIR system.
Data handling and calculation methods – Values under the limits of reporting and PFAS mass removal
A conservative approach was used while managing censored data, or data below the limit of reporting (LOR). Values below the limit of reporting were reported at the specified LOR. This method, aimed at ensuring a thorough analysis, strengthens the dependability of our results, particularly concerning data near or beneath the reporting limit.
To calculate the “mass PFAS removed” by the treatment system, influent concentrations were compared to the breakthrough curve of the effluent. The area under the resin breakthrough curve (i.e. difference in effluent versus influent concentrations) and the specific throughput (i.e. the volume treated) through the treatment system were used to determine overall PFAS mass removed.
Bench-scale resin testing
Bench-scale testing of RIEX resin samples was executed utilising schedule 40 PVC columns (height: 1.52 m; diameter: 5.1 cm). An influent flow rate of 0.65 L/min provided an empty bed contact time (EBCT) of 5 minutes, simulating the full-scale treatment system. The examined influent was sourced from the discharge from pre-treatment vessels within a similarly designed WTP located at a site in NSW, exhibiting an average PFOS + PFHxS concentration of 1 ppb. This concentration approximately simulates the PFAS concentration observed at the treatment site. The loading cycle involved 25,000 bed volumes (BVs) representing an extended duration to ensure a comprehensive assessment of the system’s performance. This performance period allowed for the observation of potential trends, breakthrough points, and any other dynamic changes that may occur during continuous operation. Samples were systematically collected at intervals of 2,500 BVs and submitted for TOC and PFAS analysis.
Figure 1: a) (top graphic) Block flow Diagram for RIEX system with b) (bottom graphic) depiction of
notional ‘hub and spoke’ regeneration system set-up.
Results
Performance assessment 2019 - 2023
Figure 2 displays influent and effluent PFAS concentrations from five points in the treatment train: 1) untreated influent to the system, 2) post-pre-treatment and before the RIEX resin, 3) effluent from the lead RIEX vessel, 4) effluent from the lag RIEX vessel, and 5) effluent from the polish IX resin vessels. Influent levels of PFAS average 16 μg/L but experience peaking events exceeding 60 μg/L (Figure 2a). As noted by the vertical lines in Figure 2b, four pre-treatment media change-outs have occurred since operations began. When this occurs, the influent PFAS concentrations to the RIEX resin are reduced. The pre-treatment media is in place to protect the resin from competing ion species (e.g., NO3-, SO42-, HCO-) and any natural organic matter (NOM) in the background water chemistry. These species compete for ion exchange sites on the resin and, if present, would ultimately reduce its PFAS capacity. The pre-treatment media is not in place to remove PFAS, although this occurs. When the pre-treatment media is exchanged for virgin media, total PFAS concentrations of ~10 μg/L are reduced to ~4 μg/L. At this site, pre-treatment media change outs are scheduled in line with budget provisions and to ensure optimum competing species/NOM removal for the long term. This change-out of approximately 5600 L of pre-treatment media occurs approximately every 24 months.
Effluent from the lead RIEX vessel consistently met the treatment objectives of <0.56 μg/L for PFOA and <0.07 μg/L PFOS + PFHxS (Figure 2c, Train A data shown). Concentrations for these target compounds (PFOS, PFHxS, and PFOA) are <0.10 μg/L in all cases. The sum of all PFAS (n=28) ranged between <0.01 μg/L to 3.06 μg/L. Further PFAS removal, particularly for the shorter-chain carboxylic acids, occurs through the lag vessel (Figure 2d). The target PFAS are all <0.01 μg/L, while the sum of all PFAS (n=28) ranges between <0.01 μg/L to 1.56 μg/L. Polish media further reduces trace PFAS before discharge to the environment through a groundwater replenishment system (Figure 2d). Concentrations from the polish vessel (Figure 2d) for target PFAS were below the analytical reporting limit in nearly all cases. The highest value recorded above the LOR for the 5-year data set was 0.01 μg/L. The LOR varied during the 5-year operational period as lab sub-contracts changed periodically, but was most frequently established at 0.01 μg/L. For the sum of PFAS (n=28), concentrations ranged from <0.01 μg/L to 0.90 μg/L with an average concentration of 0.27 μg/L. Of note, the established threshold to trigger a regeneration are based upon the Health Based Guidance Values for PFOS, PFHxS and PFOA. If greater removal were desired, or if other PFAS were required to be treated, the regeneration frequency could be easily adjusted to reach lower levels than those reported here. Although additional regeneration cycles are accompanied with an increase in operational costs, the regeneration costs are ~10-20% of the replacement and disposal costs of handling and replacing spent single use IX resin. Therefore, the use of the RIEX resin provides the client with inherent flexibility and future proofing against regulation changes that may increase PFAS removal targets.
Figure 2: Influent and effluent concentrations from the example treatment system. The system operates two parallel trains, Train A and Train B. Data is shown from Train A, similar results for Train B. “Other PFAS” = (Sum of 28 PFAS analyzed – (PFOS + PFHxS + PFOA)).
Table S1, in the supplementary materials, shows effluent concentration ranges and averages in data from the different IEX resin vessels during 5 years of operation.
Discussion
Figure 3 displays both the cumulative water volume treated and the total PFAS removed by the RIEX system to-date. In addition to removing more than 22.2 kg of PFAS from the site since operation began (Figure 3a), the continuous slope (Figure 3b) is indicative of consistent performance with nearly 1.8 billion litres treated through February of 2024. Although consistent slopes in both Figures 3a and 3b are evident, the slope in Figure 3b is almost constant. In general terms, reasons for variation in the mass of PFAS removed could be attributed to encountering hydrogeologic areas of greater PFAS concentration, seasonal PFAS flux variation in the subsurface or variability in the whole treatment train performance. The slight change of PFAS mass removed over time was not correlated to the two changeouts of pre-treatment media. This is to be expected, since the mass removed calculation is based on raw influent minus treated effluent, and so is insensitive to changes at stages between the inlet and discharge. Correlation to seasonal variation were explored by plotting rainfall versus the mass of PFAS removed (Figure 4). The site is based in tropical Northern Australia, and as such is strongly influenced by annual monsoon-like wet/dry seasonality, as is evident in the rainfall data. Interestingly, during the wet season, inlet PFAS concentrations increase up to seven-fold and then slowly taper off as the dry season takes hold. These significant spikes in PFAS concentration and mass removal suggest PFAS mass is readily leached from the source zone by rainfall infiltration during the wet season.
Figure 3: a) Cumulative WTP Treated Water volume (L) and b) Cumulative WTP PFAS Mass removed (g)
Figure 4: WTP Influent PFAS concentration vs local rainfall events;
during periods of greater rainfall, PFAS concentrations increase.
As REIX effluent concentrations reach the discharge threshold, a regeneration event is scheduled. To regenerate the resin, the relevant vessel is isolated from the treatment process, physically removed from the plant by forklift with the resin in-situ, and trucked to the regeneration facility to be run through the batch regeneration process. An additional, stand-by vessel is then placed into service so treatment may continue without delay.
To evaluate the consistency and efficacy of the regeneration process, the days between regeneration cycles and the mass of PFAS recovered during each cycle were calculated (Table S2). Many variables can influence the length of time between regeneration events: varying influent concentrations, age and capacity of pre-treatment media, number of significant rain events, and logistical issues (e.g., performing a change-out prior to a holiday/weekend). On average, a regeneration for a vessel occurs once every 8 months, after treating approximately 126 million litres water. Operationally, when employing a lead/lag configuration, regeneration of a lead vessel will occur at twice that rate, or approximately every 4 months.
While removing PFAS from influent water and reaching the customer’s treatment objective (i.e. the ADWG values) are the primary goals, the ultimate fate of PFAS removed from the aqueous matrix is also important. Figure 6 provides mass-balance calculations comparing the PFAS mass removed by the RIEX resin and the PFAS mass recovered during the regeneration process. On average, 330g of PFAS was removed by each vessel before requiring regeneration. The mass of PFAS recovered was determined from compositing samples throughout a regeneration cycle at equal intervals (e.g., at 25%, 50%, 75% and 100% through each BV of regenerant solution). Assuming the composite sample collected is representative of the effluent from the regeneration process, PFAS mass recovered closely matches, within 2.5%, the PFAS mass removed. Note, while recoveries are quite similar, minor modifications in the sampling protocols occurred and therefore limited the statistical tools that could be applied to regeneration operations; these results should be used as an estimation of the PFAS recovered.
No consistent trend of decreasing (or increasing) time between regeneration cycles was observed. Similarly, there is no trend associated with the mass of PFAS mass recovered per regeneration cycle. A noticeable trend would be indicative of incomplete PFAS removal during regeneration and an unremovable PFAS ‘heel’ building up over time. This would result in decreased resin capacity with each subsequent regeneration event. During the 5 regeneration events, data stayed fairly consistent with the exception of regeneration #3. Data for other vessels did not have similar outliers. The overestimation of PFAS recovered during this cycle may have been caused by capturing a spike of PFAS released during regeneration thereby biasing the mass recovery high.
From an operational perspective, no obvious degradation in resin performance is observed. From a physical perspective, after 5 years of regeneration cycles, no obvious breakdown in the polystyrenic bead is observed. Analysis of aged media from operational vessels was compared to new media using a high-magnification camera and infrared spectroscopy. Overall, all media appeared remarkably similar under the camera. Clear borders were visible and no evidence of brittle material indicative of breaking beads was noted. Further analysis using a scanning electron microscopy should be pursed to further validate this finding. The beads were then crushed to enable infrared spectroscopy. Multiple points on the crushed media were analyzed and the observed spectrum did not display significant variations. Figure S2 and S3 show examples of the microscopy images and IR spectrum of the analysed media.
Once the PFAS is removed from the resin and captured in the still bottoms, the current practice involves loading the PFAS onto a bed of mixed adsorbents with extended EBCT. This process is referred to as “SuperLoading”. Effluent from the SuperLoaders™ is an aqueous solution with moderate salinity, TOC and residual PFAS concentrations in the order of 10’s to 100’s ppb for sum of PFAS (n=28). At the treatment site, this solution is further processed through a stand-alone treatment train utilising single use IX media to achieve relevant environmental discharge limits. The media used in the SuperLoaders eventually becomes exhausted and is sent for disposal (generally treatment via a modern, licensed thermal desorption facility).
Figure 5: Historical look at regeneration events from March 2019 – Sept 2022 for one of four regenerable resin vessels. No trend of decreasing operational time between regeneration events is noted which would be indicative of loss of resin capacity due to incomplete regeneration or the building up of a residual PFAS heel remaining on the resin.
Waste minimisation
Waste volumes are reduced in this process as the need to discard spent resin is minimised via reuse of regenerable media; however, waste is not eliminated entirely. For example, SuperLoader™ media requires disposal on average once a year, with each replacement generating ~500kg of waste. Notably, for a given 12-month period, the three WTPs serviced by the central regeneration plant treat ~580ML of water combined, resulting in more than a million to 1 reduction in waste mass. Additionally, pre-treatment media requires replacement once exhausted. Since commissioning, approximately ~9,000 kg of pre-treatment media has required disposal. Other consumables requiring disposal include small volumes of IEX media used in distillation polishing (~100 kg). In total, accounting for all media disposal from the WTP and regeneration plant approximately 12,000 kg of combined media, have left the site for disposal. For comparison, without regenerable resin, spent media would require replacement with virgin media once the IEX capacity is reached. To date 26 vessels have been regenerated since plant start-up, avoiding cost of an additional ~26,000 L of virgin resin and disposal of ~21,000 kg of waste.
Economics
The economics of the RIEX resin system were compared to alternative treatment methods, specifically single use IX resin. In the case of RIEX resin, the avoided costs of disposing of spent media and replacing it with fresh resin are balanced by the additional costs incurred installing and operating regeneration technology. Indicative infrastructure, installation costs, and civil work required for regeneration are listed in Table 3; the costs associated with handling spent media from single use IX systems is also presented.
Table 3: Economics of regenerable resin system compared to single-use resin system.
It’s important to note that the Regeneration costs listed in Table 3 are not solely attributed to this site, since the regeneration system services two other PFAS WTPs in the area as the central regeneration hub in a ‘hub and spoke’ model (Figure 1b). As such, the cost of regeneration can be divided among the three WTP ‘spokes’ for the purposes of cost comparison with single use media/other technologies.
Figure 6 demonstrates the efficiency gains when sharing a single regeneration facility among multiple treatment sites. Although RIEX payback is achieved using a regeneration facility servicing one treatment system (at ~14 years), significant savings are realised using the hub and spoke model. When one regeneration hub services 3 treatment systems, payback is achieved in slightly over 5 years compared to an alternative of using single use resin.
Figure 6: Cost comparison for one regeneration system serving multiple PFAS-impacted sites. Costs comparison is for single-use media replacement vs the regeneration of media and capital costs of a regenerable facility. Costs do not include operational costs affiliated with single use or regenerable resin treatment systems and were assessed using the changeout criteria of managing PFOS+PFHxS concentrations to less than the HBGVs of 70 μg/L.
Optimisation and future proofing
Today, further optimisation work continues focusing on resin capacity for PFAS while maintaining the efficiency of regeneration. Recent bench-scale work using SBA resins with different functional groups has demonstrated significant improvements in PFAS capacity. Figure 7 shows results from experiments comparing the capacity of the RePURE media (currently used at the site) to an alternative media, RePURENext.
The RePURENext media consistently demonstrated more capacity than RePURE resin. Increased capacity results in less regeneration events and therefore, reduced OPEX. When comparing the BVs to 10% breakthrough for the carboxylates, the RePURENext media exhibiting ~2.7x the capacity compared to RePURE media (Figure 7a). The RePURENext media showed even greater improvements in capacity for PFAS sulfonates, demonstrating greater than 4x the capacity compared to the RePURE media (Figure 7b).
Figure 7: PFAS capacity comparisons between RePURE media used in the current treatment system and the experimental RePURENext media. a) comparison of throughput capacity for PFAS carboxylates and b) throughput capacity for PFOS + PFHxS.
Another important factor to consider with regenerable resin is the regeneration efficiency of the media. Current efforts are underway to optimize the regeneration process with the RePURENext media.
A final consideration for the use of RIEX is the concept of future-proofing. As PFAS regulation and policy evolve, having regenerable resin enables targeting of less hydrophobic and harder to remove PFAS with minimal process changes. Similarly, lower regulatory limits for the currently regulated PFAS may be created. Regulations in Europe have begun to target shorter-chain PFAS with lower hydrophobicity and earlier media breakthrough compared to longer chain PFAS. For example, Belgium regulates all PFAS to 20 ng/L including both PFHxA and PFBA. While these short-chain carboxylate PFAS are treated effectively with both RIEX and single use resin systems, the media’s capacity is reached sooner. If short-chain PFAS treatment is a priority, the RIEX system would be able to achieve these treatment objectives by increasing the regeneration frequency. With greater media use rates, the economics of RIEX compared to a single use alternative are further improved per Figure 8, which shows a shorter timeframe to breakeven for all ‘hub and spoke’ scenarios seen in Figure 7, where PFOS and PFHxS drive compliance/management decisions.
Figure 8: Payback curves demonstrating economic response to potential future regulations of PFHxA. Costs comparison is for single-use media replacement vs the regeneration of media and capital costs of a regenerable facility. Costs do not include operational costs affiliated with single use or regenerable resin treatment systems and were assessed using the changeout criteria of managing PFHxA concentrations to less than 100 ng/L.
Conclusion
Regenerable ion exchange resin has been used since 2017, effectively and efficiency removing PFAS from impacted water. In this paper, we have explored one particular system in operation since March of 2019. Over 1.8 B L of water have been treated to customer defined objectives of the ADWG values and over 22.2 kg of PFAS have been removed from the site. Consistent and exceptional up-time of the WTP has been maintained throughout the system’s lifetime and no degradation in performance has been observed over 26 regeneration cycles. Economics of the regenerable system involve increased up-front costs for regeneration equipment; however, when considering costs associated with disposal of spent media and purchase of new media, the life-cycle costs become advantageous for the RIEX system - especially when the cost of the regeneration system is distributed across 3-4 proximal WTPs. As shown, the economics of RIEX systems improve further with more stringent regulations targeting short-chain carboxylate PFAS. Additional, but hard to quantify, benefits include improved ESG metrics due to the reuse of resin, reduced future liability with significantly reduced waste generation, and the RIEX technology’s future-proof ability to meet more stringent treatment objectives with little to no process changes. Optimisation efforts continue with new media demonstrating improved PFAS capacity. Efforts are underway to optimize the regeneration of this new media. This will result in reduced operational costs for ongoing treatment to avoid adverse effects to human health and the environment.
Acknowledgements
Special Thanks to Dr. Julia Jager of Eurofins for analysis and consultation regarding the visual and infrared spectroscopy analysis of resin samples.
The Authors
David M Kempisty
Dr. Kempisty served 22 years in the US Air Force with a PhD degree in Civil Engineering. He was the Environmental Engineering Program Director at the Air Force’s graduate school, where he edited two books on PFAS. Now in industry, Dr. Kempisty provides a diverse understanding of today’s environmental issues.
Charlie Gordon
Charlie studied Environmental Engineering at RMIT and since has gained over 16 years industry experience across all facets of the contaminated land assessment and remediation project life-cycle, including in consulting, contracting and client-side roles across Australia.
After completing a Bachelor of Science at the University of Queensland, Jessica gained industry experience in Quality Management and in varied laboratory roles in research and product quality control. At ECT2, her process data analysis role focuses on compliance and optimizing treatment plant performance.
Omid Mowla
Omid brings over 14 years of industry and academic experience to ECT2, leading R&D efforts in Australia since 2021. Previously, he was a postdoctoral fellow for 4 years at the Newcastle Institute for Energy and Resources. Omid holds a Ph.D. in Chemical Engineering and is an accredited professional Environmental Engineer.
Michael G. Nickelsen
Mr. Nickelsen has over 34 years of experience in environmental investigation, remediation, and restoration technologies. He specializes in chemical oxidation and synthetic adsorbent treatment technologies. Mr. Nickelsen is an inventor on 18 process patents and has authored 40 scientific papers in peer reviewed professional journals.
Steve Woodard
Steve is the Chief Innovation Officer at Montrose Environmental Group and co-founder of ECT2, an equipment company focused on developing and commercialising treatment technologies for emerging, difficult-to-treat contaminants. Steve’s responsibilities include: leading research and new product development; providing technical leadership on all projects; intellectual property; and communication with key stakeholders.
References
Australia Government Department of Health. (2018). PFAS Expert Health Panel – Report to the Minister. Retrieved from https://www.health.gov.au/news/expert-health-panels-independent-pfas-advice (accessed June 27, 2023).
Australian Government Initiative – Water Quality Australia (2019). National Health and Medical Research Council – Retrieved from https://www.waterquality.gov.au/guidelines/drinking-water (accessed May 24, 2024).
Backe, W.J., Day, T.C., Field, J.A. (2013). Zwitterionic, cationic, and anionic fluorinated chemicals in aqueous film forming foam formulations and groundwater from U.S. military bases by nonaqueous large-volume injection HPLC-MS/MS. Environmental Science & Technology, 47(10), 5226-5234. https://doi.org/10.1021/es3034999
Baghirzade, B.S., Zhang, Y., Reuther, J.F., Saleh, N.B., Venkatesan, A.K., Apul, O.G. (2021). Thermal regeneration of spent granular activated carbon presents an opportunity to break the forever PFAS cycle. Environmental Science & Technology, 55(9), 5608-5619. https://doi.org/10.1021/acs.est.0c08224
Bolto, B., Dixon, D., Eldridge, R., King, S., Ling, K. (2002). Removal of natural organic matter by ion exchange. Water Research, 36(20), 5057-5065. https://doi.org/10.1016/S0043-1354(02)00231-2
Boronow, K.E., Brody, J.G., Schaider, L.A., Peaslee, G.F., Havas, L., Cohn, B.A. (2019). Serum concentrations of PFASs and exposure-related behaviors in African American and non-Hispanic white women. Journal of Exposure Science & Environmental Epidemiology, 29, 206-217. https://doi.org/10.1038/s41370-018-0109-y
Centers for Disease Control and Prevention (CDC). (2018). National Center for Health Statistics (NCHS). National Health and Nutrition Examination Survey Data. Hyattsville, MD: U.S. Department of Health and Human Services,
Centers for Disease Control and Prevention. Retrieved from https://cdc.gov/exposurereport/data_tables.html (accessed June 27, 2023).
Cousins, I.T., Johansson, J.H., Salter, M.E., Sha, B., Scheringer, M. (2022). Outside the safe operating space of a new planetary boundary for per- and polyfluoroalkyl substances (PFAS). Environmental Science & Technology, 56(19), 11172-11179. https://doi.org/10.1021/acs.est.2c02765.
Crone, B.C., Speth, T.F., Wahman, D.G., Smith, S.J., Abulikemu, G., Kleiner, E., Pressman, J.G. (2019). Occurrence of per- and polyfluoroalkyl substances (PFAS) in source water and their treatment in drinking water. Critical Reviews in Environmental Science and Technology, 49(24), 2359-2396. https://doi.org/10.1080/10643389.2019.1614848.
Deng, S., Yu, Q., Huang, J., Yu, G. (2010). Removal of perfluorooctane sulfonate from wastewater by anion exchange resins: effects of resin properties and solution chemistry. Water Research, 44(18), 5188–5195. https://doi.org/10.1016/j.watres.2010.06.038
Dixit, F., Barbeau, B., Mostafavi, S.G., Mohseni, M. (2021). PFAS and DOM removal using an organic scavenger and PFAS-specific resin: trade-off between regeneration and faster kinetics. Science of The Total Environment, 754, 142107. https://doi.org/10.1016/j.scitotenv.2020.142107
Dixit, F., Dutta, R., Barbeau, B., Berube, P., Mohseni, M. (2021). PFAS removal by ion exchange resins: A review. Chemosphere. https://doi.org/10.1016/j.chemosphere.2021.129777.
Emery, I., Kempisty, D., Fain, B., Mbonimpa, E. (2018). Evaluation of Treatment Options for Well Water Contaminated with Perfluorinated Alkyl Substances using Life Cycle Assessment. The International Journal of Life Cycle Assessment. https://doi.org/10.1007/s11367-018-1499-8.
EPA. (2024). Interim Guidance on the Destruction and Disposal of Perfluoroalkyl and Polyfluoroalkyl Substances and Materials Containing Perfluoroalkyl and Polyfluoroalkyl Substances – Version 2. https://www.epa.gov/system/files/documents/2024-04/2024-interim-guidance-on-pfas-destruction-and-disposal.pdf (accessed May 26, 2024).
EPA. (2022). Enforcement Alert, Violation may put ski wax users at risk from illegal perfluoroalkyl substances. EPA document # 305S21001. https://epa.gov/system/files/documents/2022-01/pfassskiwax.pdf (accessed June 27, 2023).
EPA. (2022). Drinking Water Health Advisory: Perfluorooctanoic Acid (PFOA). EPA Document Number: EPA/822/R-22/003. https://epa.gov/system/files/documents/2022-06/interim-pfoa-2022.pdf (accessed June 27, 2023).
EPA. (2022). Drinking Water Health Advisory: Perfluorooctane Sulfonic Acid (PFOS). EPA Document Number: EPA/822/R-22/004. https://epa.gov/system/files/documents/2022-06/interim-pfos-2022.pdf (accessed June 27, 2023).
EPA. (2020). Technical Brief: Per- and Polyfluoroalkyl Substances (PFAS): Incineration to Manage PFAS Waste Streams. https://www.epa.gov/sites/default/files/2019-09/documents/technical_brief_pfas_incineration_ioaa_approved_final_july_2019.pdf (accessed June 27, 2023).
Gagliano, E., Sgroi, M., Falciglia, P.P., Vagliasindi, F.G.A., Roccaro, P. (2020). Removal of poly- and perfluoroalkyl substances (PFAS) from water by adsorption: role of PFAS chain length, effect of organic matter and challenges in adsorbent regeneration. Water Research, 171, 115381. https://doi.org/10.1016/j.watres.2019.115381.
Glüge, J., Scheringer, M., Cousins, I.T., DeWitt, J., Goldenman, G., Herzke, D., Lindstrom, A.B., Lohman, R., Ng, C.A., Trier, X., Wang, Z. (2020). An overview of the uses of per- and polyfluoroalkyl substances (PFAS). Environmental Science: Processes & Impacts, 22(12), 2345-2373. https://doi.org/10.1039/d0em0029g.
Lay, D., Keyte, I., Tiscione, S., Kreissing, J. (2023). Check your tech – A guide to PFAS in electronics. EU Horizon 2020 Research and Innovation Programme. Project No. SA22171340. Retrieved from http://chemsec.org/app/uploads/2023/04/check-your-tech_230420.pdf (accessed June 27, 2023).
Levchuk, I., Marquez, J.J.R., Sillanpaa, M.; (2018). Removal of natural organic matter (NOM) from water by ion exchange – A review. Chemosphere, 192, 90-104. https://doi.org/10.1016/j.chemosphere.2017.10.101.
Liu, Y.L., Sun, M. (2021). Ion exchange removal and resin regeneration to treat per- and polyfluoroalkyl ether acids and other emerging PFAS in drinking water. Water Research, 207, 117781. https://doi.org/10.1016/j.watres.2021.117781.
Morales-McDevitt, M.E., Becanova, J., Blum A., Bruton, T.A., Vojta, S., Woodward, M., Lohmann, R. (2021). The air that we breathe: neutral and volatile PFAS in indoor air. Environmental Science & Technology Letters, 8, 897-902. https://doi.org/10.1021/acs.estlett.1c00481.
Murray, C.C., Marshall, R.E., Liu C.J., Vatankhah, H., Bellona, C.L. (2021). PFAS treatment with granular activated carbon and ion exchange resin: Comparing chain length, empty bed contact time, and cost. Journal of Water Process Engineering, 44, 102342. https://doi.org/10.1016/j.jwpe.2021.102342.
NHANES. (n.d.). Fourth national report on human exposure to environmental chemicals. Retrieved from https://www.cdc.gov/exposurereport/index.html (accessed June 27, 2023).
Salvatore, D., Mok, K., Garrett, K.K., Poudrier, G., Brown, P., Birnbaum, L., Goldenman, G., Miller, M.F., Patton, S., Poehlein, M., Varshavsky, J., Cordner, A. (2022). Presumptive contamination: A new approach to PFAS contamination based on likely sources. Environmental Science & Technology Letters. https://doi.org/10.1021/acs.estlett.2c00502.
Schellenberger, S., Liagkouridis, I., Awad, R., Khan, S., Plassmann, M., Peters, G., Benskin, J.P., Cusins, I. (2022). An outdoor aging study to investigate the release of per- and polyfluoroalkyl substances (PFAS) from functional textiles. Environmental Science & Technology. https://doi.org/10.1021/acs.est.1c06812.
Seltenrich, N. (2020). PFAS in food packaging: a hot, greasy exposure. Environmental Health Perspectives, 128(5), 054002. https://doi.org/10.1289.EHP6335.
Sen Gupta, A.K. (2017). Ion exchange in environmental processes: fundamentals, applications and sustainable technology. John Wiley and Sons, Inc. LCCN 2017016090.
Sunderland, E.M., Hu, X.C., Dassuncao, C., et al. (2020). A review of the pathways of human exposure to poly- and perfluoroalkyl substances (PFASs) and present understanding of health effects. Journal of Exposure Science & Environmental Epidemiology, 29, 131–147. https://doi.org/10.1038/s41370-018-0094-1.
Szilagyi, J.T., Avula V., Fry, R.C. (2020). Perfluoroalkyl substances (PFAS) and their effects on the placenta, pregnancy and child development: A potential mechanistic role for placental peroxisome proliferator-activated receptors (PPARs). https://doi.org/10.1007/s40572-020-00279-0.
Whitehead, H.D., Venier, M., Wu, Y., Eastman E., Urbanik, S., Diamond, M.L., Shalin A., Schwartz-Narbonne, H., Bruton, T.A., Blum. A., Wang, Z., Green, M., Tighe, M., Wilkinson, J.T., McGuinness, S., Peaslee, G.F. (2021). Fluorinated Compounds in North American Cosmetics. Environmental Science & Technology Letters. https://doi.org/10.1021/acs.estlett.1c00240.
Woodard, S., Nickelsen, M., Sinnett, M. (2018). Ion exchange for PFAS removal. In D. Kempisty, Y. Xing, & L. Racz (Eds.), Perfluoroalkyl Substances in the Environment: Theory, Practice and Innovation (pp. XX-XX). CRC Press: Taylor & Francis Group. New York. ISBN No. 9780429487125.
Woodard, S., Berry, J., Newman, B. (2017). Ion exchange resin for PFAS removal and pilot test comparison to GAC. Remediation. https://doi.org/10.1002/rem.21515.
Supplement Material
Table S1: PFAS influent (to RIEX) and effluent concentrations from Lead – Lag – Polish regenerable resin vessels. Dates of data cover 5 years of operational time from March 2019 – February 2024.
Table S2: Historical look at regeneration events from March 2019 – March 2023 for one of four regenerable resin vessels. No trend of decreasing operational time between regeneration events is noted which would be indicative
Figure S1: PFAS influent (to RIEX) and effluent concentrations from Lead – Lag – Polish regenerable resin vessels. Dates of data cover 5 years of operational time from March 2019 – February 2024.
Figure S2: Infrared spectrum of crushed virgin and used resin media. Key: red line:(RePURE virgin media; brown line: used RePURE media from lead vessel; pink line: used RePURE media from lag vessel.