Per and poly-fluoroalkyl substance (PFAS) removal by a radial flow cartridge system
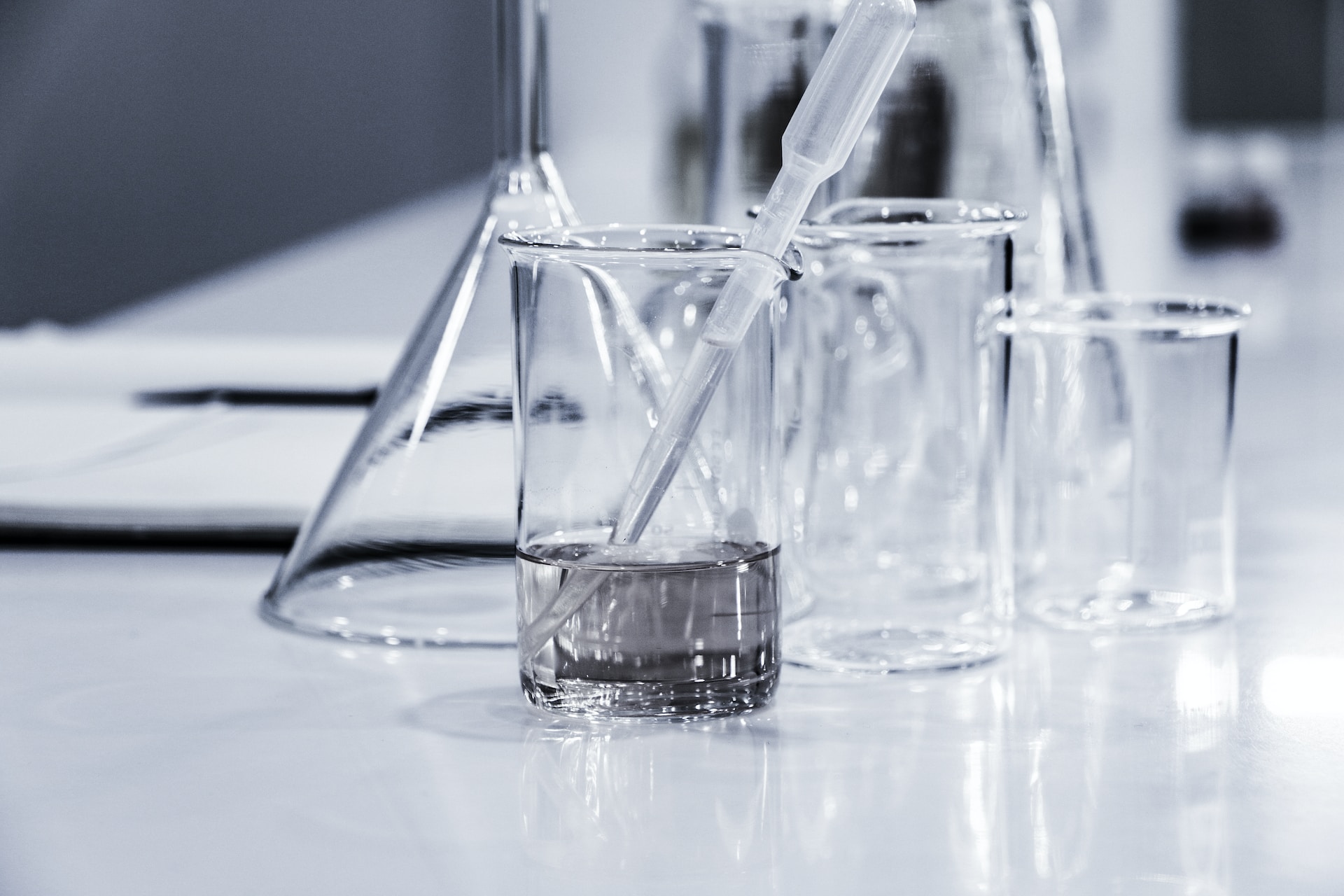
DOWNLOAD THE PAPER
Abstract
The environmental management of per and poly-fluoroalkyl substances (PFAS) is a high priority for environmental regulators around the world. There is a need for technologies that can appropriately remove PFAS from surface water. Two field-scale studies were undertaken of a radial flow cartridge system (RFCS) installed to remove PFAS from surface water at a site in Western Sydney, NSW, Australia. The RFCS incorporated a media blend for the removal of PFAS previously tested in a laboratory-scale environment by Allingham et al (2020). Results from the first field study demonstrated a PFAS removal of 87% (at a mean influent concentration of 1.43 μg/L) for a total load removal of 0.34 grams during the peak performance period – after which replacement of the RFCS media would likely be recommended to ensure high PFAS removal rates for a single RFCS. The second field study incorporated pre-treatment using a membrane cartridge system (prior to subsequent treatment by the RFCS) and demonstrated a PFAS removal of 93% (at a mean influent concentration of 66.6μg/L) for a total load removal of 2.01 grams of PFAS removed during the peak performance period. In addition to the high rate of PFAS removal provided, the RFCS is anticipated to provide additional benefits (in comparison to alternative PFAS removal technologies) that will augment the installation and operation of these systems, particularly at constrained sites, such as the ability of the system to be installed and operated below ground level.
Introduction
Background
Per and poly-fluoroalkyl substances (PFAS) are a class of manufactured chemical compounds, containing the perfluoroalkyl moiety CnF2n+1 (Buck et al 2011, Uriakhil et al 2021). Polyfluorinated PFAS also contain non-fluorinated carbon chain regions, such as –CH2-CH2-, which are known as fluorotelomers (Buck et al 2011, Uriakhil et al 2021). PFAS, fluorotelomers, and other polymers break down in the environment to form perfluoroalkyl acids (PFAAs), which are highly resistant to further degradation (Helmer et al 2021).
PFAS have a very strong Carbon-Fluorine bond (Garg et al 2021), which provides PFAS with tremendous thermal and chemical stabilities, unique wettability, and friction properties that support their role as additives in the manufacturing of flame retardants such as aqueous film-forming foams, flame-resistant materials, across the semi-electronic, packaging, anti-adhesive, and metal finishing areas (Garg et al 2021, Kotthoff et al 2015). PFAS have been produced since the 1940s and are used in various products such as aqueous film-forming foams (AFFFs), oil and water repellents, paper, and textiles (Prevedouros et al 2006). In particular, AFFFs have been used to extinguish hydrocarbon-based fuel fires at defence bases and airports which has resulted in PFAS-contaminated groundwater at these sites (Houtz et al 2013, Natarajan 2021, PFAS investigation program 2021).
PFAS, also known as “forever chemicals”, are listed as persistent organic pollutants (POPs) under the Stockholm Convention, due to their ubiquity, persistence, toxicity, and bio-accumulative nature in the environment (Jin et al 2021). This persistence has led to the bioaccumulation of PFAS in biota, aquatic and land life as well as humans (Ahrens et al 2014). Since their genesis in the 1940s, PFAS have been detected in the ambient environment, wildlife, and human serum around the globe (Blake et al 2020). Due to the widespread presence of PFAS in the environment, its unusual chemical properties, the uncertainties associated with its potential risks, and the resulting need for a precautionary approach to protect the environment and human health, the environmental management of PFAS is a high priority for environmental regulators around the world (HEPA 2020).
PFAS removal technologies
Several technologies have been developed and applied to remove PFAS from drinking water, leachate, groundwater and stormwater. These technologies collect and concentrate PFAS (e.g. via activated carbon, reverse osmosis, resins) whilst the residuals (e.g. ‘spent’ activated carbon, reverse osmosis concentrate) can be disposed of in landfills (HEPA 2020, Helmer et al 2021, Jin et al 2021, Vu et al 2022, Lath et al 2018). The removal of PFAS from within surface water (e.g. stormwater) typically involves the capture of surface water in retention dams before being pumped and treated by various technologies.
Current technologies for the removal of PFAS from surface water have high initial and ongoing costs and are generally only suitable for highly contaminated sites (with high concentrations of PFAS in ground and surface water). It is also often impractical and/ or otherwise costly to integrate these technologies given site-specific constraints (e.g. above ground line of sight restrictions for airports). There is subsequently a need for alternative PFAS removal technologies for surface water that can provide suitable removal of PFAS at a lower cost than current technologies, and can be easily integrated within constrained sites.
Radial flow cartridge system
Radial flow cartridge system (RFCS) technologies are commonly applied for the removal of pollutants from stormwater, utilising one or more media types to capture and adsorb pollutants from stormwater such as total suspended solids (TSS), hydrocarbons, nutrients, metals and other common pollutants (Dalrymple et al 2021b). The design and implementation of RFCS technologies has been developed by Contech and Ocean Protect based on over twenty years of research and development, testing and field monitoring (Dalrymple et al 2021b). Globally, over 250,000 RFCS technologies have been installed for the removal of pollutants from stormwater (Dalrymple et al 2021b). RFCS technologies can provide key advantages relative to alternative stormwater pollution removal technologies, including multiple configuration options, simple installation and management, and ability to be installed and operated underground (Ocean Protect, 2020b).
Figure 1 illustrates the components of an RFCS. Figure 2 provides an example section drawing of an RFCS installation.
Figure 1: Conceptual diagram of a single RFCS cartridge
Figure 2: Conceptual diagram of an RFCS system
The key function of an RFCS is to remove pollutants from incoming water. Any incoming water percolates through the filtration media and starts filling the cartridge central tube. The air inside the hood is purged through a one-way check valve as the water level rises in the structure. When water reaches the top of the float, buoyant forces pull the float free and allow filtered water to exit the cartridge. A siphon is established within each cartridge that draws water uniformly across the full height of the media profile ensuring even distribution of pollutants and prolonged media longevity). As the storm subsides and the water level in the structure starts falling, a hanging water column remains under the cartridge hood until the water level reaches the scrubbing regulators at the bottom of the hood. Air then rushes through the regulators breaking the siphon and creating air bubbles that agitate the surface of the filter media causing accumulated sediment to settle on the treatment bay floor. This unique surface-cleaning mechanism helps prevent surface blinding and further extends cartridge life (Dalrymple et al 2021b).
RFCS technologies are commonly applied as part of a stormwater ‘treatment train’, typically with pre-treatment technologies integrated upstream, such as ‘gross pollutant traps’ and/or ‘gully pit inserts’. These additional/ upstream stormwater treatment assets act to augment the treatment performance of the overall system by removing pollutants such as litter, particulate matter, organics and associated pollutants (e.g. heavy metals, nutrients).
An RFCS has the ability to operate with a variety of media options. To date, a total of three (3) media blends have been developed and applied in RFCS technologies – PhosphoSorb™, ZPG™ and perlite (Ocean Protect 2020b). These have been developed to target the removal of pollutants commonly found in stormwater from urban areas and/ or of concern to regulatory authorities, including suspended solids and phosphorus (Ocean Protect 2020b).
Radial Flow Cartridge System for PFAS removal
Study authors have developed a media blend for use within existing RFCS technologies to target the removal of PFAS in PFAS-contaminated ground and surface water. This media blend has been previously tested in a laboratory environment and demonstrated to achieve high PFAS removal rates (Allingham et al 2020). It is recognised, however, that laboratory-scale testing of water treatment technologies may not be representative of ‘real world’ conditions.
For PFAS performance testing, in particular, results may vary due (at least in part) to variability of coexisting inorganic and organic compounds in water matrices applied in the testing (Vu et al 2020). Highly hydrophobic dissolved organic carbon (DOC) may desorb the already-adsorbed (i.e., chromatographic effect) lower hydrophobic PFAS (e.g. short-chained ones) (Park et al 2020). In most cases, the higher presence of organic compounds resulted in decreased PFAS removal (Vu et al 2020, Marshall 2020). Furthermore, a ‘stand alone’ treatment system often fails to achieve high PFAS removal efficiencies, especially for short-chained PFAS, and a ‘treatment train’ has been recommended to augment PFAS removal (Vu et al 2020). The PFAS removal performance of an RFCS targeting PFAS removal in the ‘real world’ may, in particular, benefit from pre-treatment of incoming water to remove pollutants (e.g. solids, organic carbon) that that may be otherwise adsorbed to the RFCS media in preference to PFAS and subsequently reduce the PFAS removal and/ or longevity of the RFCS system.
Study objectives
The objective of this study was to assess the performance of an RFCS with the media blend previously developed for the removal of PFAS and tested in a laboratory-scale environment by Allingham et al (2020). The assessment included two (2) field-scale studies and focussed on two specific performance aspects: (i) ability of the RFCS to remove PFAS and (ii) assessment of lifespan (or ‘peak performance period’) of the RFCS media (i.e. time until observed PFAS removal declines, and prior to which replacement of the RFCS media would likely be recommended to ensure high PFAS removal rates).
Methotology
Site
The site of the field-scale studies is in Western Sydney, New South Wales, Australia (hereafter referred to as ‘the site’). Fire-fighting foams containing PFAS contaminants has been previously applied at the site. PFAS concentrations in surface water at the site is currently being monitored by the New South Wales Environmental Protection Agency.
Surface water runoff from the site is directed to a lined, open surface water body (referred to as the ‘primary retention dam’), where surface water is stored and treated as required. The primary retention dam has a total volume of approximately 1.6ML. Surface water from the primary retention dam was pumped to the test facility.
Study scenarios
The assessment included two (2) field-scale study scenarios:
- Study 1: Removal of PFAS from PFAS-contaminated water by a RFCS.
- Study 2: As per study 1, but with a membrane cartridge system (MCS) providing pre-treatment of water (prior to treatment by the RCFS) and with higher influent PFAS-concentrations (relative to study 1).
Treatment system & monitoring
The treatment system included an influent tank, RFCS, and effluent holding tank. As noted above, study 2 included a MCS to provide pre-treatment of PFAS contaminated water prior to subsequent treatment by the RCFS. All parts of the treatment system were enclosed.
The influent tank was an enclosed tank with a 15kL capacity, which received surface water from the primary retention dam via a submersible pump with a pre-filter. An Octave Ultrasonic water meter recorded flow rates into the influent tank. A Flomec T-series flow meter was positioned after the influent tank to record volume and flowrate entering into the treatment train. No connection contained PTFE tape. This tank was filled to capacity, and pumping then stopped prior to each assessment period.
For study 1, water from the influent tank was conveyed via a constant pressure pump to the RCFS. A ball and needle valve was used to control flows to the RCFS at a rate of 7.5 gallons per minute (monitored using one inch Flomec TM Series flowmeter). This flow rate was selected as this value is identical to the treatment flow rate of the RCFS of 7.5 gallons per minute.
For study 2, water from the influent tank was conveyed via a constant pressure pump to receive pre-treatment from a Jellyfish® MCS. The Jellyfish® is a proprietary MCS that utilises membrane filtration cartridges with high filtration surface area and flow capacity, typically integrated below ground (within an underground chamber). A Jellyfish® MCS with a 27-inch long cartridge was used, with a design treatment flow rate of 7.5 gallons per minute. The key objective of the MCS was to remove solids that may otherwise reduce the ability of the RFCS media to absorb PFAS from water. Identical to the first field-scale study, a ball and needle valve was used to control flows to the MCS at a rate of 7.5 gallons per minute. Effluent from the MCS discharged to an adjacent chamber containing an RFCS.
The chamber containing the RCFS had under-drainage (and associated false floor) to receive RFCS effluent. The RFCS was a single 460mm StormFilter® technology with a bed volume of seventy (70) litres. The StormFilter® is a proprietary RFCS comprised of one or more structures that house rechargeable, media-filled cartridges that trap particulates and adsorb pollutants from stormwater runoff. The RFCS applied a media blend developed by study authors for the removal of PFAS from surface water and tested in a laboratory scale environment by Allingham et al (2020).
Treated effluent from the RFCS discharged to a 900mm x 900mm stormwater pit used as an intermediate bulk container tank prior to being pumped via a submersible pump to a 15,000 litre effluent holding tank. The holding tank discharged to the adjacent primary retention dam.
The bed volume of treated water was defined by the volume of the RFCS (being 70 litres, as described above).
For study 1, a total of 336,000 litres of water (4800 bed volumes) was conveyed through the treatment system. The treatment system was operated and monitored in May 2021 and August 2021. During operation, one litre grab samples of water every 200 bed volumes (every 14,000 litres) from (i) influent to RFCS; and (ii) effluent from RFCS. Samples from a total of twenty four (24) events were collected and preserved, with the date and time and volume of water was recorded for each sample. Selected samples were then sent to University of New South Wales Water Quality Laboratory for analysis.
For study 2, a total of 41,790 litres of water (597 bed volumes) were conveyed through the treatment system. The treatment system was operated and monitored on 17 and 18 November 2022, and 1 and 16 December 2022. During operation, auto-samplers sampled 900mL of water every twenty five (25) minutes from (i) influent to membrane cartridge system; (ii) effluent from membrane cartridge system/ influent to RFCS; and (iii) effluent from RFCS. Samples from a total of fifty nine (59) events were collected and preserved, with the date and time and volume of water was recorded for each sample. Selected samples were then sent to ALS (a National Association of Testing Authorities accredited laboratory) for analysis. Based on the estimated peak performance bed volume (based on an extrapolation of results from study 1), a selection of the preserved samples was subsequently sent to ALS to assist in determining the peak performance bed volume of the RFCS. Samples from a total of seventeen (17) events were sent to ALS for analysis.
PFAS concentrations in all water samples were determined using a solid phase extraction (SPE) sample preparation and clean-up step followed by separation by ultra-high performance liquid chromatography and identification and quantification by tandem mass spectrometry (UHPLC-MSMS). Isotope dilution was employed to quantify target analytes with reporting limits of 0.0005 to 0.02 μg/L.
Figures 3 and 4 provide a plan and section view of the treatment system and associated monitoring at the site for study 2, noting study 1 was identical to study 2 with the exception of the Jellyfish® MCS not being present for study 1. Figure 5 provides a photo of the treatment system and associated monitoring at the site for study 2.
Figure 3: Plan view of treatment system & association monitoring at site for study 2
Figure 4: Section view of treatment system & association monitoring at site for study 2
Figure 5: Photo of treatment system & associated monitoring at site for study 2
Comparison to guideline levels
For the purposes of this study, PFAS concentrations observed within samples have been compared against Australian Drinking Water Guideline values provided by Australian Government Department of Health (2011) and HEPA (2020), which recommend a maximum value of 0.07μg/L for the Sum of PFOS and PFHxS and a maximum value of 0.56μg/L for PFOA.
Results and Discussion
PFAS removal
Figures 6 to 8 illustrate the results for PFAS concentrations and removals for study 1, including Sum of PFAS, Sum of PFOS and PFHxS, and Sum of PFOA. Figures 9 to 11 illustrate the results for PFAS concentrations and removals for study 2.
Figure 6: Study 1 results for Sum of PFAS concentrations & removals
Figure 7: Study 1 results for Sum of PFOS and PFHxS concentrations & removals
Figure 8: Study 1 results for PFOA concentrations & removals
Figure 9: Study 2 results for Sum of PFAS concentrations & removals
Figure 10: Study 2 results for Sum of PFOS and PFHxS concentrations & removals
Figure 11: Study 2 results for PFOA concentrations & removals
The study 1 results demonstrate that removal rates for PFAS declined after approximately 4,000 bed volumes, which equates to 280,000 litres of treated water (with a mean influent concentration of 1.43μg/L) and a total PFAS influent load removal of 0.34 grams. A similar trend was observed for concentrations of PFOS and PFHxS, and PFOA. This decline in PFAS removal concentrations is indicative of the capacity of the media within the RCFS to absorb PFAS reaching its limit of peak removal efficiency – commonly referred to as the ‘peak performance period’ of the treatment system, after which PFAS removal reduces. Table 1 provides a summary of the PFAS removal performance for this RCFS ‘peak performance period’ (of approximately 4000 bed volumes).
Table 1: Summary of PFAS removal performance for RFCS ‘peak performance period’
for study 1 (4000 bed volumes)
The study 2 results demonstrate that removal rates for PFAS declined after approximately 467 bed volumes, which equates to 32,700 litres of treated water (with a mean influent concentration of 66μg/L) and a total PFAS influent load removal of 2.01 grams. A similar trend for study 2 (relative to study 1) was observed for concentrations of PFOS and PFHxS, and PFOA. The MCS present for study 2 provided no significant removal of PFAS. Table 2 provides a summary of the PFAS removal performance for this RCFS ‘peak performance period’ (of approximately 467 bed volumes) for study 2.
Table 2: Summary of PFAS removal performance for RFCS ‘peak performance period’
for study 2 (467 bed volumes)
The results demonstrate that the RFCS achieved high removal rates of PFAS for both studies. The PFAS removal observed during the peak performance period for study 2 (of 93%) is slightly higher than that observed in study 1 (of 87%). The higher removal rates for study 2 are likely at least in part to the higher influent concentrations, noting it is easier for pollution removal technologies to achieve higher pollutant concentration reduction rates when influent concentrations are higher (Neumann et al, 2010). The higher removal rates for study 2 may also be due to the pre-treatment provided by the Jellyfish® MCS, described further below.
For both studies, higher removals of PFSAs were observed relative to PFCAs. There also appeared to be some desorption of PFCAs after the peak performance period in both studies, likely due to the replacement by the more hydrophobic, long-chained PFSAs and dissolved organic carbon. These observations are consistent with other large-scale studies of PFAS treatment technologies (McCleaf et al 2017, Appleman et al 2014).
For both studies, the PFOA concentrations within the RFCS effluent were below the Australian drinking water guideline maximum recommended values provided by Australian Government Department of Health (2011) and HEPA (2020), which recommends a maximum value of 0.56μg/L – although the influent POA concentrations for study 1 were also below this recommended maximum value. The Sum of PFOS and PFHxS concentrations within the RFCS effluent for both studies were, however, above the Australian drinking water guideline values provided by Australian Government Department of Health (2011) and HEPA (2020), which recommend a maximum value of 0.07μg/L.
To achieve this recommended maximum for PFOS and PFHxS concentrations for both study scenarios, RFCS technologies would need to be placed ‘in series’ to provide a multiple pass treatment system. Given the small footprint of the given RFCS (diameter of 550mm), the integration of RCFS technologies in series should be practical at many sites. Alternatively (or in addition to an ‘in series’ system), the StormFilter® RFCS is commercially available as a larger system with approximately 50% more media volume (Ocean Protect 2020b), which would further augment higher PFAS removal rates.
PFAS concentration removal rates would likely decrease after any StormFilter® RCFS integrated ‘in series’ given that, as noted above, it is easier for treatment technologies to achieve higher pollutant concentration reduction rates when influent concentrations are higher (Neumann et al, 2010). Subsequently, PFAS removal rates for each subsequent RCFS would be expected to decrease (relative to any upstream RFCS). However, it should be noted that the study 1 results demonstrated high PFAS removal rates despite significantly lower PFAS influent concentrations (relative to study 2).
It is anticipated that replacement of the media within the RFCS would be recommended prior to the end of the peak performance period to ensure high PFAS removal efficiencies. The frequency of media replacement would obviously, however, be dependent on site characteristics – particularly, the PFAS loads discharged to the RCFS. This is evidenced by the difference peak performance period between both studies (of 4,800 and 467 bed volumes for study 1 and 2 respectively), which would be largely due to the difference in influent PFAS concentrations (mean of 1.43 and 66.64 μg/L for study 1 and 2 respectively). It is subsequently anticipated that performance monitoring may be required to ensure that the ongoing treatment performance of the system was appropriate.
Treatment train
As noted above, PFAS contamination and its associated removal in real world situations is increasingly complicated due to the co-existence of inorganic and organic species (Park et al, 2020). Therefore, a stand-alone treatment often fails to effectively remove PFAS, especially short-chained PFAS, and a ‘treatment train’ is needed to solve the problem (Vu 2020).
The application of the MCS for study 2 to pre-treat untreated water (prior to subsequent treatment by the RFCS) is anticipated to augment PFAS removal by the RFCS. Whilst, as noted above, the MCS provided no significant removal of PFAS, studies of other ‘real world’ MCS applications have demonstrated high removal rates for solids and nutrients (Imbrium Stems Corporation 2012, Goonetilleke et al 2017, Kelly et al 2018). The MCS is subsequently anticipated to provide some removal of solids (including organic carbon) that may otherwise reduce the PFAS treatment performance of the RFTT.
This ‘treatment train’ approach for other sites could also include additional (or alternative) pre-treatment technologies and/ or (as noted above) additional RFCS technologies ‘in series’ for further removal of PFAS – particularly to augment the removal of any short-chained PFCAs that may desorb in an upstream RFCS due to the replacement by the more hydrophobic, long-chained PFSAs and organic carbon. This ‘treatment train’ approach would provide enhanced treatment but also possibly extend the peak performance period (and reduce the frequency of replacing RFCS).
Application
In addition to the demonstrated PFAS removal benefits of the RFCS (or MCS and RCFS ‘treatment train’) investigated as part of this assessment, the given treatment system is anticipated to provide a range of benefits in comparison to alternative PFAS removal technologies. In particular, both the MCS and RFCS technologies are both widely applied and readily understood, installed, operated and serviced technologies within Australia and overseas (Dalrymple 2021a, Dalrymple 2021b) – although additional health and safety precautions will likely be required given the human health risk concerns associated with PFAS (and sites with known or possible PFAS contamination). Both technology types are also typically installed underground (in enclosed chambers with access for suitably qualified personnel only) with minimal land requirements (Dalrymple 2021a, Dalrymple 2021b), which will be favourable for sites with above ground constraints, such as airports and military bases with line of sight constraints. Both technology types also require minimal operating hydraulic head (Ocean Protect 2021a, Ocean Protect 2021b) for the treatment of incoming water (noting both are typically installed to passively treat stormwater flows with no pumping), which will reduce or possibly avoid pumping requirements.
Conclusion
Two field-scale studies of an RFCS technology have demonstrated high removal rates of PFAS from PFAS contaminated surface water. Results from the first field study demonstrate a PFAS removal of 87% (at a mean influent concentration of 1.43 μg/L) for a total load removal of 0.34 grams during the peak performance period – after which replacement of the RFCS media would likely be recommended to ensure high PFAS removal rates for a single RFCS. Results from the second field study which, incorporated pre-treatment of PFAS contaminated water using a MCS technology (prior to subsequent treatment by an RFCS technology) demonstrate a PFAS removal of 93% (at a mean influent concentration of 66.6μg/L) for a total load removal of 2.01 grams of PFAS removed during the peak performance period.
The Authors
Brad is a Principal Environmental Engineer at Ocean Protect and Adjunct Lecturer at Griffith University. Brad has over twenty years’ experience in various projects associated with environmental engineering, and has CPEng and RPEQ accreditation.
Since January 2019, Blake has been heavily involved in the design, implementation, operation and management of a range of stormwater treatment performance monitoring programs for Ocean Protect.
Michael has owned Ocean Protect for over a decade and leads the business’ technical direction and oversees research and development. He is responsible for new product development and managing the engineering design team at Ocean Protect ensuring cost-effective environmental outcomes for developments.
Warren Jones
Warren Jones is the Lead Engineer and Engineering Manager at Ocean Protect. Warren plays a key role in shaping Ocean Protect’s stormwater field testing capabilities, providing expertise from design and implementation through to collection and analysis.
James McDonald
James McDonald is Fellow in the School of Civil and Environmental Engineering’s Water Research Centre (WRC) at the University of New South Wales.