Calculating the Hydraulic Efficiency of a Constructed Wetland
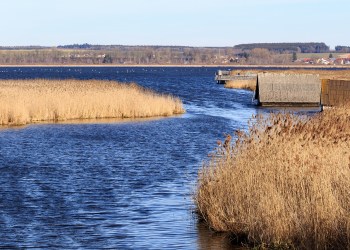
Using Rhodamine-WT to track water flow through a constructed wetland in a Mediterranean climate.
DOWNLOAD THE PAPER
Abstract
Measurement of the efficiency of constructed wetlands is critical for maintenance of their functions and contribution to improving water quality in urban areas. A fluorescent dye tracer, Rhodamine-WT, was used to track water flow through a stormwater-reliant, free water surface (FWS) constructed wetland in a Mediterranean climate. The mean hydraulic residence time (HRT) was calculated and compared to the design HRT, in order to gain an appreciation of the hydraulic efficiency of the wetland system. The actual HRT (4.0 days) was calculated to be less than the design parameters (7.0 days), giving the system a hydraulic efficiency of 0.57. Water movement was also tracked across the wetland profile to determine movement patterns within the system and identify any areas of short circuiting.
A remediation design was then implemented and tested under experimental conditions. The remediation design increased the HRT from 4.0 to 5.6 days. This equates to a hydraulic efficiency of 0.80, which indicates good performance. Improvement to the flow patterns within the wetland was also demonstrated, with the influence of short circuits reduced. Nonetheless, further changes need to be implemented within the wider wetland area to encourage even distribution of water.
Wetland processes are highly time dependent, therefore the ability to measure and maintain the efficiency of constructed wetlands is essential. This study demonstrates it is possible to improve the hydraulic residence time of a constructed wetland through changes to vegetation and obstructions to flow. It also demonstrates the benefits of dye tracer studies in tracking the lateral movement of water through the constructed wetland. The use of dye tracers is a cost-effective and valuable method to measure the hydraulic parameters of a constructed wetland and should be incorporated into the ongoing management plan for stormwater-reliant free water surface constructed wetlands.
Introduction
Constructed wetlands are a popular contemporary response to supplementary water treatment in developed areas (Greenway 2003, Ghermandi et al. 2007, Chen 2011, Scholz 2011). They can be designed to effectively treat a range of water quality issues including wastewater, stormwater, greywater and landfill leachate; while the additional values of habitat and greenspace are considered advantageous in highly urbanised catchments (Ghermandi et al. 2007, Bratieres et al. 2008, Scholz 2011). As a result, constructed wetlands are increasingly incorporated into new and existing urban developments to intercept and treat stormwater to acceptable standards prior to reuse or discharge into the environment (Greenway 2003, Chen 2011).
A constructed wetland is essentially a biological reactor with contaminant removal pathways which are highly time dependent (Werner & Kadlec 1996). Each component of the treatment wetland performs a variety of physical and biogeochemical functions; including filtration, sorption, settlement, photolysis, volatilisation and biodegradation (DeBusk 1999, Keefe et al. 2004). One of the key measures of treatment efficacy is the hydraulic retention time (HRT) of the system (Kadlec 1994). The HRT is a measurement of how long water remains within the wetland receiving treatment, and is the basis for initial wetland design. If water moves too quickly through the wetland it will not achieve its water treatment objectives (Kadlec 1994, Dieberg et al. 2005, Su et al. 2009).
While constructed wetlands are accepted as being effective at treating low-level contaminated water, there are several factors influencing the effectiveness of treatment wetlands (Holland et al. 2004, Bratieres et al. 2008, Hunter 2013). Wetlands are highly dynamic systems (Greenway 2003, Giraldi et al. 2009, Carelton & Montas 2010). The ongoing processes of vegetation development and sediment deposition result in temporal and spatial changes across the wetland profile, particularly in mature systems (Holland et al. 2004). An increase in friction due to vegetation density in one location diverts flow as the influent seeks the path of least resistance. These diversions act to exacerbate existing velocity profile and dispersion factors (Holland et al. 2004, Jenkins & Greenway 2005). If preferential flow paths develop within a system, short-circuiting may occur whereby a portion of influent moves through the wetland faster than the nominal detention time of the system (Persson 2000, Hunter 2013). As fast-moving aliquots have less time to interact with treatment surfaces they depart the wetland with little chemical alteration, potentially not meeting the desired treatment standards (Persson et al. 1999, Persson 2000). When ingrained, these diversions can isolate areas of the wetland that do not interact with flow, therefore reducing the effective volume of the treatment wetland. The degree to which actual HRT varies from design values is referred to as the hydraulic efficiency (λ) and is a direct representation of the effectiveness of the treatment system (Werner & Kadlec 1996).
The two key factors that define HRT are volume and velocity, which are determined through the initial design process (Holland et al. 2004, Su et al. 2009). However, mature wetlands accumulate vegetation and sediment over time, reducing the effective wetland volume and hydraulic efficiency (Chen 2011). The ongoing management of treatment wetlands, and the ability to manipulate HRT, is therefore important to ensure the wetland is meeting treatment objectives. In the case of existing treatment wetlands, the simplest and most cost-effective way to influence hydraulic efficiency is through the installation of flow-diversion structures (Su et al. 2009, Scholtz 2011).
To gain a better understanding of the influence of obstructions to flow on constructed wetlands, the aim of this study is to calculate the mean hydraulic residence time (HRT) of water within a constructed wetland and compare this to the design HRT to gain an appreciation of the hydraulic efficiency of the wetland system. Water movement is also tracked across the wetland profile to determine movement patterns within the system and identify any areas of short circuiting. A remediation design will then be implemented and tested under experimental conditions to determine improvement, if any, of wetland efficiency. The results lead in to discussion of suitable remediation techniques for constructed wetland systems in highly urbanised catchments.
Materials and methods
A dye tracer study was performed using Rhodamine-WT fluorescent dye (RWT) to calculate the benchmark hydraulic efficiency of an urban constructed wetland (Point Fraser wetland) in Perth, Australia. Dye tracers are highly soluble and therefore the dye molecules mimic the movement and dispersion of water molecules (Keefe et al. 2004, Arsnow et al. 2010). Rhodamine-WT is considered a conservative tracer in specific conditions, and is widely used in hydraulic studies as it resists adsorption and is detectable at low concentrations (0.1 mg/L) (Smart & Laidlaw 1977, Wilson et al. 1986, Yu-Chen Lin et al. 2003, Keefe et al. 2004). The primary method of degradation is photochemical decay however this has been shown to be negligible within the first five (5) days (Wilson et al. 1986, Yu-Chen Lin et al. 2003). Background ion concentrations can affect RWT adsorption and therefore analysis (Keefe et al. 2004), so to minimise this influence the product was mixed into ambient stormwater. When added to the wetland inlet at a known volume and constant rate, the extent of dilution measured at the outlet can be used to calculate the total system volume (Wilson et al. 1986).
One hundred millilitres of Rhodamine-WT fluorescent dye (RWT), 21.33%, was mixed with 4,500 L of recycled stormwater to a concentration of 4.74 mg L-1. The dilution was added to the inlet structure of the wetland, a vertical riser, at approximately 250 L min-1 (1,185.0 mg dye min-1). Once the dye entered the wetland it was diluted to a concentration of 9.10 ppb. The wetland auto-fill mechanism was then initiated at a rate of 0.03 m3 sec-1 for 48 hours to simulate induced flow conditions comparable to the catchment runoff for a 1-ARI event (Syrinx 2003). This ensured the flow through the system better illustrated water distribution patterns across the wetland profile.
A total of 16 transects, positioned approximately perpendicular to the expected flow of water, with sample locations every 5 metres, were used to measure water movement throughout the system (Figure 1). Samples were collected in 50 mL amber glass bottles to prevent photodegradation and returned to the laboratory for analysis at room temperature (22-27°C). Two clear glass aquaria filled with wetland water and RWT at the predefined concentration were left in situ in Zones 1 and 2 of the wetland to give baseline data for photodegradation and sorption losses.
Sample collection from the transects was timed to obtain three key readings: (1) immediately when the dye plume became observable in the transect, (2) during the peak of the visually observable plume and (3) once the dye plume had passed. After this, the sampling frequency was set to four-hourly for the first 48 hours, then twice daily thereafter. Samples were also collected at the wetland outlet with an ISCO 6712 auto- sampler (Unidata), 4-hourly for the first 3 days after dye introduction, and every 6 hours thereafter. Sampling was triggered when water depth exceeded the height of the discharge pipe (Lund et al. 2010). Samples continued to be collected from the outlet daily for a period of 2 weeks after the dye tracer was added.
Samples were analysed using a Hydrolab dataSonde 5 submersible probe and Rhodamine-WT sensor. System water without dye was used for a blank to check the change in fluorescence compared to the standard. A 3-point calibration was conducted using standard dilutions of 0 ppt (deionised water), 10 ppt and 100 ppm (Wilson et al. 1986, Hach Company 2004, Lepot et al. 2014). Mean dye concentrations for each location were overlaid on aerial imagery to highlight the preferential flow patterns in the wetland. The time series collected at the wetland outlet was used to determine the mean hydraulic residence time (HRT) and the residence time distribution (RTD). The nominal HRT was then calculated (wetland volume divided by the mean inflow velocity) and compared to the mean HRT in order to assign a measure of hydraulic efficiency (λ).
Upon analysis of the data from the dye tracer, run in conjunction with consideration of the wetland morphology, a remediation design was proposed (Figure 1). Sandbags were used to block known short circuit paths, and channels were cut into dense vegetation at a 45° angle to the expected flow path, to encourage water flow into the densely vegetated areas and increase the travel time of the influent. Water flow patterns throughout the wetland were compared to the previous results, as was the hydraulic residence time (HRT) and hydraulic efficiency (λ), to determine if a significant improvement had taken place.
Figure 1: Point Fraser Wetland, east Perth, Western Australia. Remediation design trialed under induced flow conditions at Point Fraser wetland, 29 June – 12 July 2016. Pink dashed line indicates channeling within dense vegetation, pink polygon indicates location of sandbagging and blue arrows indicate the anticipated route of water flow throughout the wetland.
Results
Baseline data
The time series created from the auto-sampler under baseline conditions did not demonstrate a clear peak in dye concentration at the outlet (Figure 3). The line of best fit indicated more than one peak, with the highest concentration of 2.9 ppm occurring on 1 August 2015, three days into the sampling. Using the trapezoidal rule, the mean HRT was estimated at 4.0 days and the mean volumetric flow rate through the wetland at 0.51 m3 hour-1. During this sampling period the concentration of dye was diluted from 485.2 ppm at the inlet to 1.9 ppm at the outlet, indicating a total wetland volume of 1,149.2 m3. This is 40% less than the design volume of 1,939.0 m3. The flow rate was calculated as 63.85 m3 sec-1. by multiplying the dye injection rate by the dilution factor (Wilson et al. 1986).
Figure 2: Volume of simulated flow (m3), plus total rainfall (mm) and total evaporation (mm) recorded for the Perth metropolitan area during field work at Point Fraser wetland, 29 July – 11 August 2015 (BOM 2015d).
Figure 3: Baseline residence time distribution (RTD) at Point Fraser wetland. Concentration of Rhodamine-WT fluorescent dye (ppm) recorded by the auto-sampler, 29 July – 7 August 2015 (SE = 0.03).Mean Rhodamine-WT concentrations at each sample location demonstrated the water distribution patterns throughout the wetland. All sample locations throughout the wetland recorded mean dye concentrations higher than background fluorescence levels. Statistically significant results indicated a preferential flow path through transect D, and between transects I-J and O (Figure 4).
Figure 4: Baseline dispersion profile at Point Fraser wetland. Mean Rhodamine-WT concentration for each sample location 29 July – 6 August 2015. Letters identify transects and arrows show direction of sampling. (All mean concentrations were above the background fluorescence levels of 1.7 ppm).
Remediation design
The time series created from the auto-sampler after implementation of the remediation design did not demonstrate a clear peak in dye concentration at the outlet (Figure 5). The line of best fit indicated more than one peak, with the highest concentration of 2.6 ppm occurring multiple times between the 4th and 11th of July, the first peak occurring six days into the run. The mean HRT was estimated at 5.6 days; an increase of 38.4 hours when compared to baseline data.
The concentration of dye was diluted from 429.5 ppm at the inlet to 1.8 ppm at the outlet, indicating a total wetland volume of 1,073.8 m3. This is 45% less than the design volume of 1,939.0 m. The flow rate, calculated by multiplying the dye injection rate by the dilution factor, was 59.65 m3 sec-1.
Figure 5: Volume of simulated flow (m3), plus total rainfall (mm) and total evaporation (mm) recorded for the Perth metropolitan area during field work at Point Fraser wetland, 29 June – 12 July 2016 (BOM 2016).
Figure 6: Residence time distribution (RTD) after remediation at Point Fraser wetland. Concentration of Rhodamine-WT (ppm) recorded by the auto-sampler; and rainfall (mm) received in the Perth metropolitan area during sampling at Point Fraser wetland, 29 June – 12 July 2016 (SE = 0.03).
Mean Rhodamine-WT concentrations demonstrated water flow throughout the wetland under induced flow conditions, with all sample locations in the wetland recording mean dye concentrations higher than background fluorescence levels (Figure 7). The remediation design demonstrated a change in the preferential flow paths with more than one primary flow path indicated in transects E, J and K; and no primary flow path indicated for transects F – H and L – M (Figure 5). The preferential flow path at transect D was not evident under the remediation design.
Figure 8 shows a comparison between the time series collected by the auto-sampler at the wetland outlet, both before and after the remediation design was implemented. The mean HRT for the remediation design is 5.6 days compared to 4.0 days for baseline conditions. The remediation design experiences the peak Rhodamine-WT concentration approximately 30 hours after baseline conditions. Analysis of the two time series indicates a significant difference (α=0.05) between sample means.
Figure 8: Comparison of dye concentration at outlet of Point Fraser wetland. Time series created during baseline conditions and after implementation of the remediation design, under induced flow conditions, at Point Fraser wetland (SE = 0.03).
Discussion
Despite natural succession towards decreased efficiency, changes can be made to the physical components of the wetland to influence flow, increase effective treatment volume and therefore improve wetland performance (Matthews et al. 1997, Dierberg et al. 2005, Su et al. 2009). Exclusion zones are arguably the easiest factor to control, through the manipulation of wetland morphology. The chosen remediation design used sandbags to block the known short-circuit paths and cut channels into dense vegetation to encourage the flow of water (Figure 1). These temporary measures should ideally be translated into permanent changes within the wetland, through inclusion in the scheduled maintenance plan, although this will probably add to the cost of ongoing wetland management.
A comparison of Rhodamine-WT concentration from the inlet and outlet of Point Fraser wetland under benchmark operating conditions produced an effective wetland volume of 1,149.2 m3. When compared to the design value of 1,939 m3, this represents a volumetric efficiency of 0.59 indicating a declining, yet satisfactory performance (Persson et al. 1999). Persson (1999) suggests that wetlands with a λ < 0.50 can still provide effective treatment due to initial overdesign, where the wetland is over-sized for the anticipated catchment runoff volumes. This is typically to buffer against temporal and spatial changes within the wetland (Le Coustumer et al. 2008). Nonetheless, overdesign can have serious management implications in Mediterranean climates, particularly for lined stormwater-dependent systems; and may result in an over-dependence on irrigation water for top-up during the summer months (Masi & Martinuzzi 2007).
The effective volume of the wetland, as calculated by dye dilution for the remediation design, was 1,073.8 m3. This represents a volumetric efficiency of 0.55, which is less than the volumetric efficiency calculated during baseline conditions (0.59). Standard error for both sets of data are comparable (SE = 0.03) and considered acceptable for the small range of concentrations (range = 1.3); however, this result was unexpected. Factors influencing the effective volume of the remediation design include the volume and positioning of sandbags which may have isolated problematic areas of the wetland. Other variables include timing, as the dye tracer run for the remediation design was conducted 12 months after the baseline dye tracer run, and conditions within the wetland in terms of vegetation and sediment which may have changed significantly.
Residence time distribution
In an ideal, well mixed system, dye will appear at the outlet after the nominal retention period, reach peak concentration and then decrease exponentially (Persson et al. 1999). The reality is far more complex. The residence time distribution (RTD) curve of a constructed wetland is valuable as it provides an indication of flow patterns and can be used to calculate the mean HRT of the system (Kadlec & Knight 1996, Holland et al. 2004). Polynomial regression analysis of the RTD under baseline conditions demonstrates more than one peak in dye concentration (rcalc>rcrit, α=0.05), with the highest concentration of 2.9 ppm occurring three days into the sampling period. The multiple peaks may suggest additional flow paths through the wetland (Persson et al. 1999) or may simply represent pulse events caused by changed flow conditions (Figure 2).
Polynomial regression analysis of the RTD for the remediation design also suggested more than one peak in dye concentration (rcalc>rcrit, α=0.05). However, in contrast to baseline conditions, the peak concentration of 2.6 ppm occurred on multiple occasions over the course of a week; with the first peak occurring six days into the run (compared to three days). Once again this indicates several paths through the wetland, but comparison of the RTD for baseline and remediation design indicates that the peak Rhodamine-WT concentration increased by approximately 30 hours when compared to baseline data (Figure 8).
Hydraulic efficiency
The hydraulic efficiency (λ) of a wetland is a measure of the system’s ability to treat influent to required standards and is calculated by comparing the design HRT to the actual HRT (Werner & Kadlec 1996). The design HRT for Point Fraser is 7.0 days. A minimum retention time of 72 hours can be achieved using a manual release valve for anything greater than a 1-ARI event (Syrinx 2003).
The mean HRT under baseline conditions was 4.0 days and represents a hydraulic efficiency (λ) of 0.57. In this instance, the mean HRT cannot be compared to the minimum retention time of 72 hours, as the total of rainfall plus autofill volume delivered during this period did not exceed a 1-ARI event (Figure 2). The value of λ is comparable to the volumetric efficiency, indicating a declining, yet satisfactory performance (Persson et al. 1999). Nonetheless, there were clear preferential flow paths visible in the dispersion profile (Figure 4).
The mean HRT was calculated after implementation of the remediation design as 5.6 days, an increase of 38.4 hours when compared to baseline conditions. The hydraulic efficiency also increased substantially from 0.57 under baseline conditions to 0.80, demonstrating good performance. However, analysis of variance between the two data sets indicated that the probability of error was equal to the variation between the two samples (fcalc=fcrit, α=0.05) and a larger sample is required to validate these results.
Dispersion profile
The friction associated with morphological features of the wetland causes velocity and dispersion effects and is demonstrated to influence the overall efficiency of the system (Holland et al. 2004). Therefore, to demonstrate the patterns of water movement throughout the wetland, mean Rhodamine-WT concentrations for each sample location were mapped along transects to create a dispersion profile.
The vegetated biofilter separates the first zone of the wetland into two open pools. Its role is to filter suspended sediment and promote even water distribution across the wetland profile (Syrinx 2009). The dispersion profile under baseline conditions (Figure 4) clearly demonstrates this is not the case. The dispersion profile demonstrates a distinct and statistically significant preferential flow path through transect D, effectively allowing influent to avoid the vegetated biofilter entirely. Under baseline conditions the biofilter inhibited water from moving through the wetland.
This indicates that the vegetated biofilter may not be fully utilised during natural and low flow events. Sediment may potentially be funneled into the second open pool of the wetland, resulting in reduction of volume over time. Furthermore, the uneven deposition of sediment may cause barriers which isolate or impede flow (Holland et al. 2004). Sediment-bound contaminants including phosphorus and heavy metals (Ekholm & Lehtoranta 2012) may pass through the first zone of the wetland ahead of design prediction (Persson 2000, Hunter 2013). Other common issues exacerbated by lack of flow include stagnating areas, increased water temperatures, excess algae growth and reduced dissolved oxygen levels (Wong et al. 1999).
The dispersion profile for baseline conditions indicates that the influent makes its way into the second zone of the wetland via the low point of the weir and separates into two key flow paths (Figure 4). The most dominant flow path showed the influent being diverted into a series of open pools which have developed over time, in order to avoid the resistance associated with emergent vegetation (Holland et al. 2004, Jenkins & Greenway 2005). Densely vegetated areas of the wetland are where the majority of contaminant removal takes place. Aside from the physical process of filtration; microbial processes in the rhisosphere actively remove dissolved nutrients and contaminants from the water column in addition to those which are taken up by the plants themselves to convert into biomass (Paul & Clark 1996, Stottmeister et al. 2003, Vymazal 2007, Reddy & De Laune 2008, Hunter 2013). Dissolved carbon is also removed from the water column by wetland plants and used for cellular growth (Vymazal 2007, Bernal & Mitsch 2012). As the water avoids these important treatment areas, the effectiveness of the wetland is reduced.
Statistically significant high mean concentrations in transects I, J and O highlighted a second shortcut and provided evidence of water overtopping the soil mound during induced flow conditions (Figure 4). Once again this reduces the effective volume of the wetland, as whole sections of the wetland are not involved in the treatment process. Fast moving particles have less time to interact with treatment surfaces and may depart the wetland ahead of the minimum retention period. This can result in water leaving the wetland without meeting treatment objectives (Persson et al. 1999, Persson 2000, Holland et al. 2004).
The remediation design demonstrated a change in the preferential flow paths within the wetland (Figure 7). Sandbags used to block the open gap in transect D, combined with channelling into the vegetated biofilter successfully encouraged water flow into the biofilter and away from the short circuit. This created a wider zone of influence for the water in the first zone of the wetland, however there was still a significant portion that did not contribute significantly to water treatment. This may be because of the effects of the densely vegetated biofilter, in conjunction with the effects of gravity on slow moving water particles encouraging water towards the lower AHD areas in the open zone. Additional sandbagging in the second zone of the wetland to deflect water away from the soil mound and the open pools, combined with channelling within the densely vegetated sections had some impact, to a lesser degree. It increased the zone of influence for water across transect J and reduced overtopping of the soil mound.
Conclusions
The actual hydraulic residence time of the Point Fraser wetland is less than the design parameters (5.6 compared to 7.0 days), however it is considered to be meeting treatment objectives due to initial over-design (Persson et al. 2000, Le Coustumer et al. 2008). The remediation design increased the HRT from 4.0 to 5.6 days (Figure 8). This equates to a hydraulic efficiency of 0.80, which indicates good performance. Although the remediation design resulted in an increase in the hydraulic efficiency, an increased sample size is required to reliably validate the analysis of variance. Improvement to the flow patterns within the wetland was also demonstrated, with the influence of short- circuits reduced. Nonetheless, further changes need to be implemented within the wider wetland area to encourage even distribution of water. Supplementary planting within the wetland to maintain vegetation coverage, in addition to thinning out of particularly dense areas will be required on an ongoing basis, representing an increase to scheduled maintenance costs.
For future dye studies, use of an in-situ fluorometer would improve the accuracy of results. Manual sampling along transects caused water disturbance and likely increased the interference from organic matter in some samples, however, was considered necessary to create the dispersion profile. If manual sampling is undertaken, background fluorescence and turbidity levels should be measured before each sample collection. This will prevent error from the build-up of fluorescence over consecutive sample runs and automatically account for system losses via photolysis and sorption reactions (Lepot et al. 2004).
This study has highlighted the value of dye tracer studies to track water dispersion across the wetland profile. Observation of the mean HRT for Point Fraser before the remediation design indicated adequate performance (λ = 0.57), however the preferential flow paths highlighted by sampling across transects revealed short-circuiting and excluded areas of the wetland. Without rectification, the hydraulic efficiency would continue to decrease over time resulting in the wetland potentially not meeting treatment objectives. The remediation design demonstrated that simple and cost-effective changes to the wetland morphology can influence both hydraulic efficiency and distribution patterns, improving wetland performance. The use of dye tracer methodology can enable wetland managers to measure the efficiency of constructed wetlands in a cost-effective manner to ensure wetland function is meeting the desired treatment objectives.
About the author
Shelley Harrington | Shelley has worked in various government roles for the past 13 years, including both Federal and local government, and is currently a Project Coordinator in the parks and environment space for City of Perth. Having qualifications in both Environmental Management and Marine Science, her primary interests relate to wetland management, as these ecosystems represent the connectivity and continuum between land and water. Shelley’s ultimate goal is to be engaged in life-long learning, in a role which continues to develop her skills and experience in the technical field of environmental science, and deliver real results for the community.