Biomimetic membrane technology developments relevant to sustainable reuse of resources
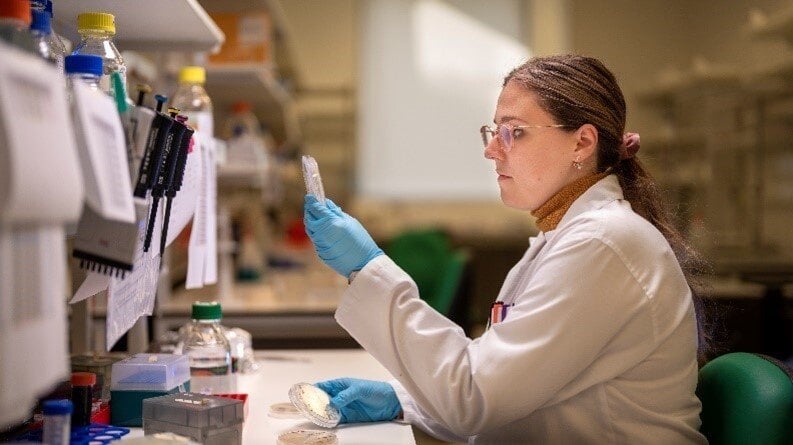
Abstract
Membrane technologies are increasingly sought to provide solutions for processes in industrial and municipal wastewater management, manufacturing, and intensified agriculture systems that were traditionally met through natural ecosystem services provided by plants and microbes in lower-intensity systems. Services like water filtration and nutrient recycling, energy conversion and generation are under increasing demand from growing urban populations and intensive agriculture. Scalable, economically viable technologies are needed to capture and recycle critical resources which are often lost in current, linear treatment pathways of complex or high-volume waste streams. This type of membrane technological advancement needs to occur, while also preserving the biodiversity of plants and microbes in their native environments, to reduce the burden that human activities place on Earth’s natural ecosystems. Specifically, biomimetic membranes that incorporate selective proteins such as aquaporins are discussed as an emerging technology that has great potential to selectively separate valuable resources from complex liquid media or wastewaters.
Introduction
Shifting from linear to circular resource use
Industries and governments are increasingly recognising the risks posed by the current linear model of “take-make-use-dispose” processes. A circular economy is an alternative resource management model that aims to decouple food, energy and material production from the consumption of finite resources. It operates on three principles: “design out waste and pollution; keep products and materials in use; and regenerate natural systems” (Ellen MacArthur Foundation, 2019). Water plays multiple roles as a “resource, nutrient carrier, source of energy and service”, and enabling water to contribute these roles is a function that can be mirrored in water and wastewater treatment processes (WSSA, 2021). Water utilities and other industries have roles as “agents for the circular economy” and as “resource stewards” of nutrients, water and energy (Figure 1). Both water and nutrients are limited resources which can ideally be recaptured and reused with innovative water management systems (Jazbec, Mukheibir and Turner, 2020).
Figure 1. Achieving sustainability and development ambitions requires adoption of circular economy systems. This involves continuous flow of materials, where products and materials are kept in circulation through processes such as reuse, repair, remanufacturing and recycling, and ensuring nutrients from biodegradable materials are returned to the Earth to regenerate nature (Arsic et al., 2022; Ellen MacArthur Foundation, 2023).
Identification of functional markets for circular products is a key step in transitioning to a circular economy. For example, the beneficial reuse of nutrient-rich by-products separated from liquid and solid waste streams that water utilities manage can create circular value chains. The need for nutrient rich fertilisers has grown with increasing demand for food and textiles, particularly the requirement for essential plant nutrients nitrogen and phosphorus (Schröder et al., 2010). The production of nitrogen fertilisers has a high energy and greenhouse gas footprint, consuming 1-2% of global energy production and accounting for 1.2% of global anthropogenic carbon dioxide emissions (Soloveichik, 2019; Kyriakou et al., 2020) and phosphorus is a finite mineral resource that can experience as much as 80% losses between “mine to fork” (Schröder et al., 2010; Zou, Zhang and Davidson, 2022). It has been estimated that globally, wastewaters contain 16.6 Mt of nitrogen and 3 Mt of phosphorus, and if recovery and reuse was prioritised, this could offset approximately 13% of global nutrient demand from fertilisers (Qadir et al., 2020). Technologies can be developed to create effective and viable circular products that keep resources in use. For example, designing technologies such as selective membranes to specifically remove phosphorus or nitrogen species in a liquid form could meet both regulatory limits for the wastewater utility and also produce a clean stream of nutrients that could be utilised more easily by agricultural end-users.
Discoveries and Innovations
Roles of membranes in nature
Biological membranes underpin all life on Earth (Figure 2). Without membranes, life as we know it would not have evolved. Membranes enable the separation of different chemistry on the inside of cells relative to the outside, and they support maintenance of an electrical potential difference across the membrane. It was the formation of biological membranes that enabled the first living cells to have the capacity to capture and manage key resources and undertake the chemical reactions required for life (Kurihara et al., 2015). The presence of microbial cells can be traced back more than 3.2 billion years (Javaux, Marshall and Bekker, 2010; Dodd et al., 2017).
Figure 2. Plant and microbial activities sustain life. Everything alive requires a cell membrane. Living cell membranes enable plant and microbial organisms to generate and recycle the raw materials needed to deliver important resources (Ozaki and Reinhard, 2021) and services, like food, feed, fibre, oxygen, water filtration, carbon capture, solar radiation absorbance, nutrient recycling and climatic cooling services. In the present, relative to 3000 years ago, the collective function of plants and microbes in recycling raw materials has been handicapped due to the impacts of human activities that destroyed many of the functional ecosystems that were delivering resource recycling services. Membrane biotechnologies can contribute to supporting a sustainable future. By learning from how natural ecosystems achieved circular resource recycling services it is possible to develop technologies that can contribute to lifting a portion of the burden that human activities place on the natural environment.
Without exception, life requires energy and water, and membranes are required to utilise and manage these resources. Membranes influence which molecules enter and leave cells and they play essential roles in maintaining and converting between chemical and electrical energy. Living cell membranes contain proteins that contribute to their unique function. These proteins are encoded by genes in living organism genomes. Some proteins are peripheral to the membrane and support chemical reactions or signalling within cells and some are integral to the membrane and have key roles in channelling or transporting molecules across the membrane. Membrane channel and transport proteins are generally highly specific to the transport of particular molecules and their function is typically tightly controlled by the cell to maintain optimum internal cell chemistry. Membrane proteins can be passive or active, some transport substances down the concentration gradient, which does not require energy, others can transport against a concentration gradient, which often does require energy. In nature membrane proteins play crucial roles in controlling the movement of water, nutrients and a range of physiologically relevant molecules across cellular and subcellular membranes (David et al., 2019).
Biological membranes facilitate functions essential for the survival of every living thing because they support energy conversion and circular use of resources (Figure 2) (Ozaki and Reinhard, 2021; Westermann et al., 2023). For example, plant membranes have particularly important energy conversion roles because they contribute to converting light energy into electrochemical energy through photosynthesis (Croce and van Amerongen, 2014). In photosynthesis, the green pigment in leaves (chlorophyll) absorbs light energy from the sun, and converts this to chemical energy gradients across a membrane used to drive chemical reactions that generate sugar (plant biomass) and oxygen from CO2 and water (Huokko et al., 2021). In addition to energy conversion, biological membranes also perform efficient carbon capture services in the environment by enabling CO2 to be highly concentrated within cells, and then stored as biomass. For example, efficient carbon capture services come from membrane functions in vegetation such as mangroves (Krauss et al., 2022) and seagrasses (Green, Chadwick and Jones, 2018) in coastal estuaries (Rosentreter et al., 2023) and membranes present in the types of soils where microbial populations drive soil organic carbon accumulation (Tao et al., 2023). Biological membranes are also important for global water security and play important roles in the global water cycle (Alvarez et al., 2018). The membranes in plants facilitate transpiration, the process whereby soil water taken up by the plant is released from leaves into the air as water vapour. Transpiration from plants accounts for more than half of all terrestrial evaporation in the water cycle (Good, Noone and Bowen, 2015). Transpiration forms a critical link between the global water and carbon cycles as water loss from leaves is a necessary consequence of the carbon (CO2) capture of photosynthesis, as well as influencing ecosystem function, productivity and forming part of an optimal hydrological cycle (van Noorden, 2006). Optimal hydrological cycles supported by the influence of plant vegetation can deliver regular, moderate and productive rainfall. In the absence of the influence of plants on they hydrological cycle rainfall is more likely to be either insufficient, leading to drought, or excessive, leading to flooding, neither of which are productive. Both drought and flood are associated with poor water quality outcomes
Considering the essential services that membranes provide for life on Earth, it might be expected that preserving the natural environments that currently provide irreplaceable membrane functions would be a high priority. However, contaminated water generated by human activities is released into natural environments and it is exceeding what the natural environment is capable of filtering for us, a problem that will continue to grow alongside global population growth. Preservation of the critical services that natural environments provide involves reducing the burden that human activities inflict on natural environments. Reducing this burden involves taking responsibility for the wastes that humanity creates and investing in the development and adoption of types of technologies and activities that enable those wastes to be turned into resources that can be reused by following circular economy principles.
Taking membrane innovation cues from nature
Membranes are progressively being incorporated into resource recycling technologies and advanced energy generation and storage systems (Uliana et al., 2021). For example, in filter technologies, membranes are used to separate clean water from wastewater so that the clean water can be reused (Zhang et al., 2023). Membranes are also used to process and refine chemicals (Kurimoto et al., 2023) and gases (Tan et al., 2022) to create products and manage industrial waste, in reverse electrodialysis (RED) (Cusick, Kim and Logan, 2012), pressure-retarded osmosis (Zhu et al., 2018; Wu and Field, 2019) (Figure 3) and as componentry in advanced batteries (Zhang et al., 2023). However, the functionality of manufactured membranes can be further advanced to incorporate desirable attributes of biological membranes.
Figure 3. Membranes and their interactions with solutes facilitate conversions of energy. In living cells changes in chemical, electrical, light and thermal energy are facilitated by membranes, and in renewable energy generation technologies energy can be generated from differences in electrical potential across membranes. Key: ∆ = change in (voltage, or time). In natural ecosystems living cell membrane separation of molecules contributes to energy generation and circular use of resources. In membrane technologies membranes can contribute to energy generation and separation of target resources from complex mixtures (De Rosa et al., 2023; Zhu et al., 2023).
Biological membranes can selectively regulate the flux and separation of molecules and convert between energy forms (Figure 3). Development of innovative manufactured membrane technologies that mimic these biological features and functions can create opportunities to harvest essential limited resources from wastes (De Rosa et al., 2023). Examples of the types of services where membrane technologies have the potential to make greater contributions include:
- Selective separation of key resources like nutrients, metals, minerals and clean water from contaminated water and industrial and urban wastewater for reuse (Uliana et al., 2021; Sandru et al., 2022).
- Extraction of greenhouse gasses from the atmosphere, and energy production, like generation of green hydrogen (Sandru et al., 2022).
- Innovative new processes that generate the raw materials needed for energy, food and water security in the future (Figure 2 and 3; Zuo et al., 2023; Zaitchik et al., 2023).
Specific examples were membrane advances have been inspired by copying nature include the recent development of a crystalline carbon nitride membrane mimicking biological ion channels which is being optimised for separating lithium ions from brines, and which could support extraction of resources needed for clean energy storage systems (Zhang et al., 2024). The membrane design was inspired by highly selective biological ion channels that efficiently discriminate between different ions and this advance offers new possibilities for efficient extraction of a valuable element from brines and battery wastes. Inspiration for developing membranes for separating CO2 from air has been drawn from studying the active transport of CO2 across biological membranes (Metcalfe et al., 2024). Plant polymer based materials have also been used to create bio-inspired systems for selective removal of heavy metal contaminants from environmental water (Nakahata et al., 2024). These types of advances will be critical to meeting the increasing demand for finite resources, for creating economic value from circular systems, and for reducing the burden human activities place on the ecosystems that have traditionally provided these types of services and resources.
One of the most important features of living cell membranes is the control of water and solute transport. In all life forms, there is a type of membrane protein called an aquaporin (AQP) that contributes to controlling water transport. A Nobel Prize was awarded in 2003 to Peter Agre and Roderick Mackinnon for their studies of the human aquaporin, HsAQP1, for its role in regulating water transport in red blood cells (Agre, 2006). Many aquaporins, including HsAQP1, have a central channel surrounded by four monomeric channels. The monomeric channels of AQPs can be selective for water, while the central channel can be permeable to other solutes, like ions, and each channel can be individually opened or closed during regulation of their function (Yool, Stamer and Regan, 1996; Yu et al., 2006). Nature has created aquaporins that can transport billions of water molecules per second. Other types of aquaporins co-transport various ions, gases, nutrients, metalloids and orgaanic molecules such as sugars (Tyerman et al., 2021; Venkataraghavan et al., 2023). Incorporation of components like aquaporins into commercial membranes has the potential to deliver innovative selective features (De Rosa et al., 2023).
Development of membrane technologies
The passage of water through biological membranes was observed by Abbe Nollet in 1748 and was the first reported indication of the semi-permeability or selectivity of biological membranes. The phenomena of osmosis, the movement of solvent molecules through a semipermeable membrane from an area of lower solute concentration to one of higher solute concentration was further studied in the early to mid-1800s by Dutrochet. However, it was not until the turn of the 20th century that cell membranes were considered independent structures that can be selectively permeable. In 1902, Nagano reported membranes with different permeabilities to sugar, and the first report of a transport protein was in 1931. The field of membrane transport proteins has since grown to one of the most significant fundamental fields in biological research, influencing advances in many applied fields, such as crop improvement and medicine. For a detailed historical account of the discoveries and developments surrounding cell membranes see Lombard (2014).
Following study of the function of natural membranes in the mid-1700s researchers developed commercial membranes and membrane composites and these have been scaled to address a range of industrial applications (Figure 4). Nitrocellulose membranes were discovered in 1846 and the first synthetic nitrocellulose membranes were made in 1856. In the 1900s, techniques to make nitrocellulose membranes of graded pore size were developed. Research on dialysis and reverse osmosis progressed in the 1920s and micro porous membranes became commercially available in 1930. Research on electrodialysis continued in the 1930s along with the development and use of microfiltration technology to process drinking water around the end of World War II.
Figure 4. Examples of influential developments in membrane technology research over time. The previous technological advances that led to development of the commercial membranes available today were inspired by learning from how natural membranes function (Lombard, 2014). There is opportunity to continue to advance membrane technologies through the process of studying how natural membranes function, particularly in relation to how waste can be separated to generate resources for reuse.
The 1950s brought more breakthroughs in reverse osmosis research, and developments in gas separation on silicone rubber and pervaporation in 1957. In the early 20th century, membrane technologies were explored to address various underlying industrial separation challenges, including microfiltration (MF), ultrafiltration (UF), nanofiltration (NF), reverse osmosis (RO), membrane distillation (MD), pervaporation (PV), and gas separation. A pivotal moment arrived in 1959 with the development of the first cellulose acetate RO membrane capable of effectively separating water from brine solution. Composite membrane research progressed in the 1960s, including manipulation of pore size and development of thin-film composite membranes. This innovation featured a thin top-layer supported by a thick, highly porous substrate. As a result, RO became practical for desalination with salt rejection values reaching approximately 99.5%.
The latter half of the twentieth century brought capillary membranes, membranes with immobilised carriers and active centres, immersed membrane bioreactors, and in 2000 pervaporation was applied for large-scale applications. Various membrane module designs, including tubular, hollow fibre and spiral wound configurations were developed for commercial-scale applications, further enhancing the utility of membrane technology in diverse industrial processes. Since then, new membrane processes have been developed, such as novel gas separation and pervaporation approaches and carbon nanotube membranes. Currently, membrane technologies are benefiting from biomimicry approaches where learnings from natural membrane functions are applied (De Rosa et al., 2023).
Membrane technology development foci
Innovative developments in membrane technologies fall into categories such as materials, manufacturing, biomimicry, and hybridisation.
1. Materials: The composition of membrane lipids and supporting polymer structures in living organisms is diverse. There is great scope to learn from nature to find optimal membrane and polymer compositions and structures that improve membrane selectivity and productivity.
2. Manufacturing: The processes involved in making membranes and membrane polymer composites play a pivotal role in the efficiency, cost-effectiveness and environmental sustainability of manufactured membranes.
3. Biomimicry: Approaches that mimic functions observed in biological systems have the potential to create advanced and highly selective membranes. For example, incorporating the types of membrane proteins found in living organisms into commercial membrane technologies.
4. Hybridsation: Tailored membranes can be integrated with other materials, such as clay, graphite or metal organic frameworks, in modular, sequential systems to take advantage of the strengths of each type of material. This has potential to improve overall efficiency, increase energy use efficiency and reduce operation costs.
These types of developments are likely to enable broader adoption of membrane technologies in the future across a diverse range of industries, ultimately contributing to resource conservation and sustainable industrial practices.
Separation membranes have transitioned from scientific concept to a practical and influential technology for water purification and industrial resource separation (Figure 4). They can be environmentally sustainable, economically viable and user-friendly, and assist in the separation and recovery of valuable compounds from complex mixtures generated by industries such as petrochemical, pharmaceutical and food. Recognition of these attributes continues to inspire research into advancing membrane technologies and attracts end-user attention.
Pressure driven membrane processes are by far the most widely applied membrane technologies in reclaiming clean water from wastewater, along with broader filtration and separation application categories, such as:
- Microfiltration (MF): MF membranes have a pore size of between 0.1-10 μm. They are mainly used for removal of suspended particles, colloids and microorganisms from industrial feed streams, and are popular as a pre-treatment process for complex wastewater generated in biopharmaceutical, food and food processing industries.
- Ultrafiltration (UF): UF membranes have smaller pore sizes (0.01-0.1 μm) and are largely used for rejection of viruses, colloids and polypeptides from industrial liquids, such as from dairy, food and beverage industries.
- Nanofiltration (NF): NF membranes are thin film composite membranes containing a thin film layer (<1 μm) on top of a porous substrate (50-150 μm) for small ion separation, capable of rejecting multivalent salts and uncharged solutes, while allowing some monovalent salts to pass through. Common applications include separation of mixtures of ions and small organic molecules, water softening, concentrating or desalting, and treating of industrial effluents.
- Reverse osmosis (RO): RO membranes are similar to NF membranes but they have tighter structures capable of rejecting monovalent ions. RO is commonly used for desalination, wastewater treatment, solvent dehydration and organic-organic separation, and removal of contaminants and impurities from water to make it suitable for drinking.
Examples of challenges in optimising the function of membrane technologies include:
- Achieving high selectivity and specificity so that membranes can distinguish between different molecules or ions.
- Limiting membrane fouling and scaling, particularly in water treatment and desalination membranes; fouling occurs when suspended or dissolved materials accumulate on the membrane surface reducing performance. Scaling involves deposition of mineral salts on the membrane.
- Improving energy efficiency for pressurised membrane separation systems
- Increasing the resistance of membrane systems to harsh chemicals, high temperatures and mechanical stresses to minimise membrane degradation over time.
- Reaching scale and improving cost-effectiveness
Improvements in membrane functions that address these challenges are needed to expand the range of applications, upgrade separation efficiency and reduce running costs. In particular, options for addressing fouling and scaling for high-pressure reverse osmosis and nanofiltration applications are needed to improve the energy efficiency of these systems, and the development of more durable membranes will reduce system maintenance and replacement costs.
For wastewater treatment applications, examples of membrane performance and limitations were recently reviewed by Lee et al. (2023). One of the challenges identified was the fine control of pore sizes - an area where the study of biological membrane channels and the development of biomimetic membranes can inform research advances.
Current state and future prospects of biomimetic membranes
Biomimetic membrane research, particularly in the development of aquaporin (AQP)-based membranes, remains in the early-to-mid stages, especially with regard to large-scale commercial applications. Increasing interest among researchers is being driven by the unique advantage these membranes offer - combining high water permeability with excellent selectivity. Several successful laboratory-scale studies have been progressed over the years, and the next steps involve large-scale commercial implementation (Azarafza et al., 2023). A few pioneering companies, such as ‘Aquaporin A/S’ and ‘Mangrove’, have made significant strides in commercialising AQP-based membranes. Their work shows that AQP-containing vesicles incorporated into the selective layer of reverse osmosis (RO) and forward osmosis (FO) membranes can outperform conventional membranes in terms of water permeability and selectivity (Abaie, Xu and Shen, 2021; Reddy, Kalla and Murthy, 2022). There will be challenges faced in scaling up this technology for widespread industrial applications. These include optimising production processes, ensuring long-term membrane stability, and reducing costs to enable broader adoption.
Fabrication of biomimetic membranes poses challenges such as stabilising biological components like AQPs within synthetic membrane matrices. A major limitation is the lack of fundamental understanding of the interactions between functional protein channels and the supporting matrix materials, making the selection of appropriate fabrication materials critical (Shen et al., 2014). Challenges such as protein denaturation, membrane fouling, and the long-term stability of protein-incorporated membranes under continuous operational conditions remain significant barriers (Ryu et al., 2019). Of particular consideration is the potential for poor compatibility between AQPs and synthetic membrane components, which can result in non-selective voids that significantly reduce separation performance. These factors must be addressed to enable the successful large-scale application of biomimetic membranes in industrial processes.
There is pressing need to develop robust, scalable and cost-effective methods for integrating biomimetic components into scalable membrane systems. Opportunities for innovation include optimising membrane synthesis parameters, such as the immobilisation or incorporation of AQP-embedded vesicles, refining cross-linking processes, and controlling membrane thickness to enable large-scale production. Collaborative efforts with industry are essential to address scalability challenges while ensuring consistent membrane quality and performance across production batches and in diverse application settings.
Analysis of the techno-economic feasibility of aquaporin-based hollow fibre membranes revealed that aquaporin membranes had potential for better performance than traditional membranes. This is because their water flux, clean water recovery and contaminant rejection measures lead to competitive annualised costs (Dsilva Winfred Rufuss et al., 2024). Engineering efforts directed at optimisation of aquaporin membranes are leading to improvements in membrane resistance to fouling. For example, aquaporin nanovesicles functionalised with polyethylene glycol brushes, termed PEGylation, through a facile self-assembly approach were incorporated in the selective layer of thin-film composite membranes through interfacial polymerisation and tested (Li et al., 2023). The tests revealed that the aquaporin nanovesicle membranes functionalised with PEGylation had high water permeance (~8.2 LMH/bar) and NaCl rejection (96.4%), and relative to the unmodified aquaporin-membranes the functionalised membranes had 100% permeate flux recovery after water cleaning whereas unfunctionalised membranes had 85% permeate flux recovery after water cleaning (Li et al., 2023). This indicates that there is potential to improve the performance of aquaporin embedded membranes through modifying their surface properties, such as the hydrophilicity.
Incorporating mechanisms from living cell membranes into biomimetic membranes
Water-selective living cell membrane components, such as aquaporin proteins, have been successfully incorporated into commercial membrane separation technologies, but there may be mechanisms with other selectivity features that could be relevant to advancing membrane technologies (Li et al., 2023). Aquaporins provide multifunctional and adaptive roles in living cells and they have become building blocks for manufactured membrane materials used for filtering and separation applications (Figure 5) (Tyerman et al., 2021; Byrt et al., 2023; De Rosa et al., 2023). The Aquaporin Z protein from Escherichia coli cells was used in the development of biomimetic membranes for a range of water treatment applications (Xie et al., 2013; Górecki et al., 2020). The E. coli Aquapoin Z contains narrow channels which allow passage of single water molecules while excluding the passage of other solutes (Savage et al., 2003). This water selective mechanism relies on a combination of size exclusion, electrostatic repulsion, and dipole reorientation. In the future the incorporation of other aquaporins in membrane separation technologies that have additional transport functions could expand the applications for biomimetic membranes (De Rosa et al. 2023).
Research into incorporating diverse membrane protein mechanisms with selectivity for target molecules such as nutrients and metals into commercial membranes is in early stages. Previous research indicates that incorporation of aquaporins into membrane technologies has potential to help improve energy efficiency and selectivity (Azarafza et al., 2023; Chen et al., 2023; Li et al., 2023; Fuwad et al., 2024; Hegab et al., 2024). Since aquaporins provide a variety of selectivity functions in living cell membranes there is potential to expand their application to develop biomimetic membranes that can separate desirable or undesirable ions from mixed solutions (Figure 5). For example, aquaporins can have applications in separating plant nutrient ions, critical minerals or toxic elements from complex mixtures (Tyerman et al., 2021; Byrt et al., 2023; De Rosa et al., 2023). Membranes with these types of functions incorporated could create concentrated streams of nutrient rich water from wastewater streams or remove heavy metals from contaminated waters.
Figure 5. Representation of a common type of biomimetic membrane structure. Selective structures, such as aquaporin proteins are embedded into lipid vesicles (liposomes). The aquaporin-embedded liposomes are imprinted into a non-porous polymer layer, typically a polyamide formed through interfacial polymerisation, which is structurally supported by a porous support layer (following (Porter et al., 2020)).
Future prospects in wastewater management with biomimetic membrane technologies
In nature diverse types of living cell membranes contain nutrient-selective aquaporins which support separation of molecules that are relevant to industrial wastewater applications. For example, there are aquaporins that can separate ammonia, urea, and potassium (Kirscht et al., 2016; Qiu et al., 2020). These types of nutrient resources are valuable when available in purified forms, but they are problematic when present in wastewater because they contribute to greenhouse gas emissions. Ammonia present in wastewater runoff from agriculture and aquaculture causes environmental damage, such as waterway pollution and algal blooms, and led to nitrous oxide emissions. Nitrous oxide is both a toxin and potent greenhouse gas. As the global energy system transitions away from fossil fuels, ammonia can be used as a zero-carbon fuel for hard to abate energy applications such as heavy transportation and high temperature industrial processes, so it is worthwhile to harvest ammonia from wastewater for use in fertilisers and fuels. Ammonia is an important industrial feedstock, most critically for fertilisers to support modern agriculture, and in textile, metallurgical, and mining industries.
Study of living cell mechanisms involved in ammonia separation, and development of ammonia-selective components for integration into membrane separation technologies for extraction and reuse of ammonia resources from waste could contribute to addressing the problem of ammonia loss in wastewater. Development of new ammonia-selective membrane technologies could enable recovery of ammonia from wastewater, mitigate emissions from run-off and provide a sustainable and low emissions source of ammonia for industry and agriculture. Nature has already evolved mechanisms to selectively transport ammonia across cell membranes. By taking inspiration from nature, it is likely to that future membrane technologies could deliver scalable highly selective membranes facilitating the recovery of both clean water and ammonia from wastewater. Implementation of this type of innovative separation technologies would be expected to deliver significant environmental and economic benefits, addressing critical environmental and resource challenges and ensuring a sustainable future for water and ammonia resources. Hypothetically, incorporation of a range of nitrogen-containing nutrient molecule selective mechanisms into membrane separation technologies could enable wastewater treatment plants to extract those nitrogen containing molecules that lead to problematic nitrous oxide emissions. This principle could be extended to explore whether other resource-selective mechanisms from nature can inform development of membrane separation technologies for separating a diverse range of target resources from waste.
Exploring the intersections of membrane proteins, like aquaporins, and natural and manufactured membranes
Biological membranes are crucial components in natural ecosystems, transporting and regulating resources like nutrients, water, and energy. Mimicking functions that biological membranes deliver in commercial membranes capitalises on functions and efficiencies that nature has evolved over billions of years (Figure 2). Biomimetic, or bioinspired, membranes incorporate biological components or use concepts and ideas inspired by biology (Shen et al., 2014). For example, selective membrane proteins can be manufactured and embedded in commercial membranes, to form bio-assisted synthetic structures that mimic the selectivity and function of biological proteins (Di Vincenzo et al., 2021). Research and development of biomimetic membranes is accelerating and has potential for selective separation of resources.
Incorporation of the types of membrane proteins that have evolved in nature, like aquaporins, into innovative membrane technologies has been reported to contribute to membrane performance by improving selectivity, energy efficiency and limiting fouling (Xie et al., 2013; Chun et al., 2018). Future advances in optimising the function of manufactured membranes using mechanisms that nature has evolved will require additional innovative developments, such as:
- Large-scale fermentation systems for selective protein production to upscale protein-embedded membrane fabrication.
- Advances in areas such as ultra-centrifugation and affinity purification to improve the efficiency of extracting membrane proteins from cultures.
- Optimisation of reagents such as detergents, to provide ideal extraction conditions when purifying target proteins.
- Improved efficiency of protein integration into artificial membrane systems (Ryu et al., 2019).
- Investigation of cleaning options to prevent fouling and scaling (Giwa et al., 2017) to improve membrane durability and long-term stability, particularly where they are used in desalination.
- Introducing circular economy principles into the design of novel membranes to explore options for improved sustainability of the membranes themselves, such as considering the life cycle of the product, the ability to recycle components, and considering the impact of certain chemical or material inputs in the production of the membranes.
The field of biomimetic membrane development is evolving rapidly, and the investigation of biological systems is valuable for supporting innovation in this area. Future economic scale-up of biologically-inspired precision membrane systems are expected to contribute to efficient water purification and recovery of valuable resources from complex wastes.
Conclusion
Advancement of biomimetic membrane technologies and their integration in circular bioeconomy processes have potential to support food, fuel and material production in the face of increasing global population, a shifting climate and pressure on finite resources, including water and land. Membranes are increasingly being shown to be powerful tools to recover valuable resources from mixed liquid streams. The development of biomimetic membranes, such as aquaporin-based membranes, could enable the separation of a range of industrially important resources from wastewater including nitrogen, phosphorus, metal, contaminants and organic biochemicals. This could support the development of a range of novel, circular products to direct those useful biomolecules and nutrients back into our food, fibre and materials value chains while also capturing water for reuse and decreasing the impact of wastewaters on the environment.
Acknowledgements
This research is supported by the inaugural ANU-CSIRO Agri-Food Collaboration Program (AFCP). The team would like to thank ANU, CSIRO and the ANU Agrifood Innovation Institute for project funding and management, intellectual property management and academic mentoring support. C.S.B was supported by the Australian Research Council IM240100006. For this article, we particularly thank Natalie De Santis from ANU for referencing support. Figures created with BioRender.com
The Authors
Maja Arsic
Dr Maja Arsic is a Winanga-y Postdoctoral Research Fellow in the Sustainability Program at CSIRO. Maja has a background in plant nutrition and environmental chemistry, with a focus on phosphorus. Maja currently works at the science and policy interface to enable circular bioeconomy approaches for sustainable food systems.
Caitlin Byrt
Caitlin Byrt is a Professor at the Research School of Biology, ANU. Caitlin is a bioengineer working on developing future crops and membrane biotechnologies. The future crops that Caitlin works on are designed to be adapted to challenging environments, such as dry and saline soils. The membrane biotechnologies her team are developing are designed to enable precious metal, mineral, nutrient and clean water resources to be harvested from wastes so that they can be reused.
Annamaria De Rosa
Dr Annamaria De Rosa is a Postdoctoral Research Fellow in the Plant Sciences Division in the Research School of Biology at the Australian National University. Annamaria’s research focuses on investigating and characterising the small-scale membrane separation mechanisms naturally occurring in plant cell membranes and translating these plant-derived strategies to novel bioinspired industrial wastewater processing technologies.
Pollyanna Hilder
Dr Pollyanna Hilder is a Research Scientist at the CSIRO Agriculture and Food Aquaculture Group. Pollyanna’s work has focused on aquaculture finfish production with an emphasis on larviculture and early life history. Polly’s work has explored new aquaculture species selection, finfish reproductive physiology, larviculture with focus on the visual environment, recirculating aquaculture systems (RAS) and aquaculture resource re-use.
Shagufta Iqbal
Shagufta Iqbal is a post-doctoral researcher at the Research School of Biology, ANU. She has a background in biochemistry with a special focus on membrane transport proteins and the mechanism of transport. She is currently working with a multi-disciplinary team in developing aquaporin inspired water filtration technology.
Samantha McGaughey
Dr Samantha McGaughey is a Postdoctoral Research Fellow in the Plant Sciences Division at the Research School of Biology ANU. Her research focusses on understanding the cell membrane transport systems that are essential for plant nutrition, development and stress response.
Shiva Prasad Nandala
Dr Shiva Prasad Nandala, CERC Postdoctoral Fellow, in the Sustainability Program, CSIRO Agriculture & Food and Manufacturing. Shiva is a dedicated researcher with a profound interest in advancing membrane technology to optimize separation processes. His expertise lies in the development of innovative membranes tailored to enhance separation characteristics across diverse applications. Shiva’s work extends to fabricating membranes specifically designed for water treatment, protein separation, and gas separation.
Cathryn O’Sullivan
Cathryn is a senior research scientist in the Sustainability program in CSIRO Agriculture and Food. Her research explores the intersection between circular economy, bioenergy production and sustainable farming systems. Over the years she has explored biomethane and biohydrogen production, recovery of energy and nutrients from organic, agricultural and mixed waste streams, the role of microbes in transforming wastes into valuable products and use of novel farming systems to enable Net Zero and sustainable agriculture.
Xing Wu
Dr Xing Wu is a Research Scientist with the Advanced Materials and Processing program at CSIRO Manufacturing. Xing’s research interests center on membrane fabrication and modification for energy and environmental applications. He possesses expertise in designing high-performance membranes tailored to specific applications on various scales for desalination and wastewater treatment.
Zongli Xie
Dr Zongli Xie is a Principal Research Scientist and a Research Group Leader of Materials for Energy, Environment and Health at CSIRO Manufacturing. From membrane materials design to scale up systems, Dr Xie has worked closely with industries in advancing innovative membrane materials and emerging membrane technologies for environment and energy including desalination and wastewater treatment.
References
Abaie, E., Xu, L. and Shen, Y. (2021) ‘Bioinspired and biomimetic membranes for water purification and chemical separation: A review’, Frontiers of Environmental Science & Engineering, 15(6), p. 124. Available at: https://doi.org/10.1007/s11783-021-1412-8.
Agre, P. (2006) ‘The Aquaporin Water Channels’, Proceedings of the American Thoracic Society, 3(1), pp. 5–13. Available at: https://doi.org/10.1513/pats.200510-109JH.
Alvarez, P.J.J. et al. (2018) ‘Emerging opportunities for nanotechnology to enhance water security’, Nature Nanotechnology, 13(8), pp. 634–641. Available at: https://doi.org/10.1038/s41565-018-0203-2.
Arsic, M. et al. (2022) ‘Australia needs a national policy approach to successfully implement circular bioeconomy in agriculture and food systems’, 19, pp. 46–60.
Azarafza, A. et al. (2023) ‘Aquaporin-Based Biomimetic Membranes for Low Energy Water Desalination and Separation Applications’, Advanced Functional Materials, 33(21), p. 2213326. Available at: https://doi.org/10.1002/adfm.202213326.
Byrt, C.S. et al. (2023) ‘Exploring aquaporin functions during changes in leaf water potential’, Frontiers in Plant Science, 14. Available at: https://www.frontiersin.org/articles/10.3389/fpls.2023.1213454 (Accessed: 15 December 2023).
Chen, Y. et al. (2023) ‘Evaluation of aquaporin based biomimetic forward osmosis membrane in terms of rejection performance for contaminants in greywater and its membrane fouling properties’, Chemosphere, 333, p. 138983. Available at: https://doi.org/10.1016/j.chemosphere.2023.138983.
Chun, Y. et al. (2018) ‘Prototype aquaporin-based forward osmosis membrane: Filtration properties and fouling resistance’, Desalination, 445, pp. 75–84. Available at: https://doi.org/10.1016/j.desal.2018.07.030.
Croce, R. and van Amerongen, H. (2014) ‘Natural strategies for photosynthetic light harvesting’, Nature Chemical Biology, 10(7), pp. 492–501. Available at: https://doi.org/10.1038/nchembio.1555.
Cusick, R.D., Kim, Y. and Logan, B.E. (2012) ‘Energy Capture from Thermolytic Solutions in Microbial Reverse-Electrodialysis Cells’, Science, 335(6075), pp. 1474–1477. Available at: https://doi.org/10.1126/science.1219330.
David, R. et al. (2019) ‘Roles of membrane transporters: connecting the dots from sequence to phenotype’, Annals of Botany, 124(2), pp. 201–208. Available at: https://doi.org/10.1093/aob/mcz066.
De Rosa, A. et al. (2023) ‘Molecular membrane separation: plants inspire new technologies’, New Phytologist, 238(1), pp. 33–54. Available at: https://doi.org/10.1111/nph.18762.
Di Vincenzo, M. et al. (2021) ‘Biomimetic artificial water channel membranes for enhanced desalination’, Nature Nanotechnology, 16(2), pp. 190–196. Available at: https://doi.org/10.1038/s41565-020-00796-x.
Dodd, M.S. et al. (2017) ‘Evidence for early life in Earth’s oldest hydrothermal vent precipitates’, Nature, 543(7643), pp. 60–64. Available at: https://doi.org/10.1038/nature21377.
Dsilva Winfred Rufuss, D. et al. (2024) ‘Techno-economic feasibility of advanced aquaporin-based hollow fiber forward osmosis membrane in industrial wastewater treatment’, Journal of Water Process Engineering, 67, p. 106155. Available at: https://doi.org/10.1016/j.jwpe.2024.106155.
Ellen MacArthur Foundation (2019) It’s Time For A Circular Economy | Ellen MacArthur Foundation, It’s Time For A Circular Economy. Available at: https://www.ellenmacarthurfoundation.org/.
Ellen MacArthur Foundation (2023) What Is A Circular Economy? Available at: https://www.ellenmacarthurfoundation.org/topics/circular-economy-introduction/overview (Accessed: 21 November 2023).
Fuwad, A. et al. (2024) ‘Highly permeable and shelf-stable aquaporin biomimetic membrane based on an anodic aluminum oxide substrate’, npj Clean Water, 7(1), pp. 1–11. Available at: https://doi.org/10.1038/s41545-024-00301-0.
Giwa, A. et al. (2017) ‘Biomimetic membranes: A critical review of recent progress’, Desalination, 420, pp. 403–424. Available at: https://doi.org/10.1016/j.desal.2017.06.025.
Good, S.P., Noone, D. and Bowen, G. (2015) ‘Hydrologic connectivity constrains partitioning of global terrestrial water fluxes’, Science, 349(6244), pp. 175–177. Available at: https://doi.org/10.1126/science.aaa5931.
Górecki, R. et al. (2020) ‘Improved reverse osmosis thin film composite biomimetic membranes by incorporation of polymersomes’, Journal of Membrane Science, 593, p. 117392. Available at: https://doi.org/10.1016/j.memsci.2019.117392.
Graham, B. (2024) The Future of Food: Are Australian food companies failing nature?, The Future of Food: Are Australian food companies failing nature? Available at: https://assets.nationbuilder.com/auscon/pages/28703/attachments/original/1721606776/Future_of_Food.pdf?1721606776 (Accessed: 1 August 2024).
Green, A., Chadwick, M.A. and Jones, P.J.S. (2018) ‘Variability of UK seagrass sediment carbon: Implications for blue carbon estimates and marine conservation management’, PLOS ONE, 13(9), p. e0204431. Available at: https://doi.org/10.1371/journal.pone.0204431.
Hegab, H.M. et al. (2024) ‘3D hierarchical aquaporin-like nanoporous graphene membrane with engineered tripartite nanochannels for efficient oil/water separation’, npj Clean Water, 7(1), pp. 1–11. Available at: https://doi.org/10.1038/s41545-024-00303-y.
Huokko, T. et al. (2021) ‘Probing the biogenesis pathway and dynamics of thylakoid membranes’, Nature Communications, 12(1), p. 3475. Available at: https://doi.org/10.1038/s41467-021-23680-1.
Javaux, E.J., Marshall, C.P. and Bekker, A. (2010) ‘Organic-walled microfossils in 3.2-billion-year-old shallow-marine siliciclastic deposits’, Nature, 463(7283), pp. 934–938. Available at: https://doi.org/10.1038/nature08793.
Jazbec, M., Mukheibir, P. and Turner, A. (2020) Transitioning the Water Industry with the Circular Economy, prepared for the Water Services Association of Australia, Institute for Sustainable Futures, University of Technology Sydney, Water Services Association of Australia. Available at: https://www.wsaa.asn.au/publication/transitioning-water-industry-circular-economy (Accessed: 21 November 2023).
Kirscht, A. et al. (2016) ‘Crystal Structure of an Ammonia-Permeable Aquaporin’, PLOS Biology, 14(3), p. e1002411. Available at: https://doi.org/10.1371/journal.pbio.1002411.
Krauss, K.W. et al. (2022) ‘Mangroves provide blue carbon ecological value at a low freshwater cost’, Scientific Reports, 12(1), p. 17636. Available at: https://doi.org/10.1038/s41598-022-21514-8.
Kurihara, K. et al. (2015) ‘A recursive vesicle-based model protocell with a primitive model cell cycle’, Nature Communications, 6(1), p. 8352. Available at: https://doi.org/10.1038/ncomms9352.
Kurimoto, A. et al. (2023) ‘Bioelectrocatalysis with a palladium membrane reactor’, Nature Communications, 14(1), p. 1814. Available at: https://doi.org/10.1038/s41467-023-37257-7.
Kyriakou, V. et al. (2020) ‘An Electrochemical Haber-Bosch Process’, Joule, 4(1), pp. 142–158. Available at: https://doi.org/10.1016/j.joule.2019.10.006.
Lee, J. et al. (2023) ‘Performance, limitation, and opportunities of acid-resistant nanofiltration membranes for industrial wastewater treatment’, Journal of Membrane Science, 666, p. 121142. Available at: https://doi.org/10.1016/j.memsci.2022.121142.
Li, X. et al. (2023) ‘Engineering Ultra-Permeable and Antifouling Water Channel-based Biomimetic Membranes toward Sustainable Water Purification’, Journal of Membrane Science Letters, 3(2), p. 100049. Available at: https://doi.org/10.1016/j.memlet.2023.100049.
Lombard, J. (2014) ‘Once upon a time the cell membranes: 175 years of cell boundary research’, Biology Direct, 9(1), p. 32. Available at: https://doi.org/10.1186/s13062-014-0032-7.
Metcalfe, I.S. et al. (2024) ‘Separation and concentration of CO2 from air using a humidity-driven molten-carbonate membrane’, Nature Energy, pp. 1–10. Available at: https://doi.org/10.1038/s41560-024-01588-6.
Nakahata, M. et al. (2024) ‘Hyperconfined bio-inspired Polymers in Integrative Flow-Through Systems for Highly Selective Removal of Heavy Metal Ions’, Nature Communications, 15(1), p. 5824. Available at: https://doi.org/10.1038/s41467-024-49869-8.
van Noorden, R. (2006) ‘More plants make more rain’, Nature [Preprint]. Available at: https://doi.org/10.1038/news060925-1.
Ozaki, K. and Reinhard, C.T. (2021) ‘The future lifespan of Earth’s oxygenated atmosphere’, Nature Geoscience, 14(3), pp. 138–142. Available at: https://doi.org/10.1038/s41561-021-00693-5.
Porter, C.J. et al. (2020) ‘Pathways and Challenges for Biomimetic Desalination Membranes with Sub-Nanometer Channels’, ACS Nano, 14(9), pp. 10894–10916. Available at: https://doi.org/10.1021/acsnano.0c05753.
Qadir, M. et al. (2020) ‘Global and regional potential of wastewater as a water, nutrient and energy source’, Natural Resources Forum, 44(1), pp. 40–51. Available at: https://doi.org/10.1111/1477-8947.12187.
Qiu, J. et al. (2020) ‘Phosphorylation influences water and ion channel function of AtPIP2;1’, Plant, Cell & Environment, 43(10), pp. 2428–2442. Available at: https://doi.org/10.1111/pce.13851.
Reddy, A.S., Kalla, S. and Murthy, Z.V.P. (2022) ‘Biomimetic membranes: Advancements and applications — A minireview’, Bioresource Technology Reports, 18, p. 101047. Available at: https://doi.org/10.1016/j.biteb.2022.101047.
Rockström, J. et al. (2023) ‘Safe and just Earth system boundaries’, Nature, 619(7968), pp. 102–111. Available at: https://doi.org/10.1038/s41586-023-06083-8.
Rosentreter, J.A. et al. (2023) ‘Coastal vegetation and estuaries are collectively a greenhouse gas sink’, Nature Climate Change, 13(6), pp. 579–587. Available at: https://doi.org/10.1038/s41558-023-01682-9.
Ryu, H. et al. (2019) ‘Biomimetic Membranes with Transmembrane Proteins: State-of-the-Art in Transmembrane Protein Applications’, International Journal of Molecular Sciences, 20(6), p. 1437. Available at: https://doi.org/10.3390/ijms20061437.
Sandru, M. et al. (2022) ‘An integrated materials approach to ultrapermeable and ultraselective CO2 polymer membranes’, Science, 376(6588), pp. 90–94. Available at: https://doi.org/10.1126/science.abj9351.
Savage, D.F. et al. (2003) ‘Architecture and Selectivity in Aquaporins: 2.5 Å X-Ray Structure of Aquaporin Z’, PLOS Biology, 1(3), p. e72. Available at: https://doi.org/10.1371/journal.pbio.0000072.
Schröder, J.J. et al. (2010) Sustainable Use of Phosphorus, Plant Research International Report 357: Sustainable Use of Phosphorus. Available at: https://edepot.wur.nl/163942 (Accessed: 1 August 2024).
Shen, Y. et al. (2014) ‘Biomimetic membranes: A review’, Journal of Membrane Science, 454, pp. 359–381. Available at: https://doi.org/10.1016/j.memsci.2013.12.019.
Soloveichik, G. (2019) ‘Electrochemical synthesis of ammonia as a potential alternative to the Haber–Bosch process’, Nature Catalysis, 2(5), pp. 377–380. Available at: https://doi.org/10.1038/s41929-019-0280-0.
Tan, X. et al. (2022) ‘Truly combining the advantages of polymeric and zeolite membranes for gas separations’, Science, 378(6625), pp. 1189–1194. Available at: https://doi.org/10.1126/science.ade1411.
Tao, F. et al. (2023) ‘Microbial carbon use efficiency promotes global soil carbon storage’, Nature, 618(7967), pp. 981–985. Available at: https://doi.org/10.1038/s41586-023-06042-3.
Tyerman, S.D. et al. (2021) ‘Adaptable and Multifunctional Ion-Conducting Aquaporins’, Annual Review of Plant Biology, 72(1), pp. 703–736. Available at: https://doi.org/10.1146/annurev-arplant-081720-013608.
Uliana, A.A. et al. (2021) ‘Ion-capture electrodialysis using multifunctional adsorptive membranes’, Science, 372(6539), pp. 296–299. Available at: https://doi.org/10.1126/science.abf5991.
Venkataraghavan, A. et al. (2023) ‘Barley HvNIP2;1 aquaporin permeates water, metalloids, saccharides, and ion pairs due to structural plasticity and diversification’. bioRxiv, p. 2023.04.17.537278. Available at: https://doi.org/10.1101/2023.04.17.537278.
Westermann, L.M. et al. (2023) ‘Bacterial catabolism of membrane phospholipids links marine biogeochemical cycles’, Science Advances, 9(17), p. eadf5122. Available at: https://doi.org/10.1126/sciadv.adf5122.
World Economic Forum (2020) Nature Risk Rising: Why the Crisis Engulfing Nature Matters for Business and the Economy, World Economic Forum. Available at: https://www.weforum.org/publications/nature-risk-rising-why-the-crisis-engulfing-nature-matters-for-business-and-the-economy/ (Accessed: 1 August 2024).
WSSA, W.S.A. of A. (2021) ‘WSAA Strategy 2021-23’, Water Services Association of Australia Strategy 2021-23. Available at: https://www.wsaa.asn.au/sites/default/files/publication/download/WSAA%20Strategy%202021%20FINAL%20%285%29.pdf (Accessed: 21 November 2023).
Wu, J.J. and Field, R.W. (2019) ‘On the understanding and feasibility of “Breakthrough” Osmosis’, Scientific Reports, 9(1), p. 16464. Available at: https://doi.org/10.1038/s41598-019-53417-6.
Xie, W. et al. (2013) ‘An aquaporin-based vesicle-embedded polymeric membrane for low energy water filtration’, Journal of Materials Chemistry A, 1(26), pp. 7592–7600. Available at: https://doi.org/10.1039/C3TA10731K.
Yool, A.J., Stamer, W.D. and Regan, J.W. (1996) ‘Forskolin Stimulation of Water and Cation Permeability in Aquaporin1 Water Channels’, Science, 273(5279), pp. 1216–1218. Available at: https://doi.org/10.1126/science.273.5279.1216.
Yu, J. et al. (2006) ‘Mechanism of Gating and Ion Conductivity of a Possible Tetrameric Pore in Aquaporin-1’, Structure, 14(9), pp. 1411–1423. Available at: https://doi.org/10.1016/j.str.2006.07.006.
Zaitchik, B.F. et al. (2023) ‘Wetting and drying trends under climate change’, Nature Water, 1(6), pp. 502–513. Available at: https://doi.org/10.1038/s44221-023-00073-w.
Zhang, Y. et al. (2024) ‘Congener-welded crystalline carbon nitride membrane for robust and highly selective Li/Mg separation’, Science Advances, 10(24), p. eadm9620. Available at: https://doi.org/10.1126/sciadv.adm9620.
Zhang, Ying et al. (2023) ‘A smart risk-responding polymer membrane for safer batteries’, Science Advances, 9(5), p. eade5802. Available at: https://doi.org/10.1126/sciadv.ade5802.
Zhu, B. et al. (2023) ‘Boosting membrane carbon capture via multifaceted polyphenol-mediated soldering’, Nature Communications, 14(1), p. 1697. Available at: https://doi.org/10.1038/s41467-023-37479-9.
Zhu, X. et al. (2018) ‘Unique ion rectification in hypersaline environment: A high-performance and sustainable power generator system’, Science Advances, 4(10), p. eaau1665. Available at: https://doi.org/10.1126/sciadv.aau1665.
Zou, T., Zhang, X. and Davidson, E.A. (2022) ‘Global trends of cropland phosphorus use and sustainability challenges’, Nature, 611(7934), pp. 81–87. Available at: https://doi.org/10.1038/s41586-022-05220-z.
Zuo, P. et al. (2023) ‘Near-frictionless ion transport within triazine framework membranes’, Nature, 617(7960), pp. 299–305. Available at: https://doi.org/10.1038/s41586-023-05888-x.