Better Understanding Wastewater Treatment’s Nitrous Oxide Emissions
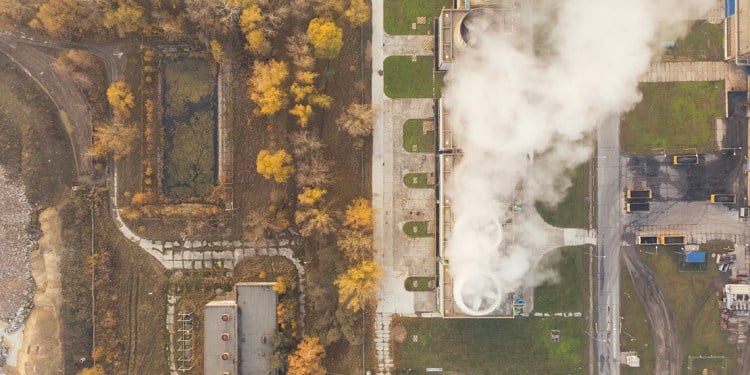
The case for revised reporting protocols using variable emission factors based on nitrogen removal
DOWNLOAD THE PAPER
Introduction
Nitrous oxide (N2O) is a major contributor to direct (Scope 1) greenhouse gas (GHG) emissions from wastewater treatment plants (WWTPs). Activated sludge systems typically represent the bulk of mainstream treatment processes for municipal WWTPs, both in Australia and most developed countries. N2O has been measured in the off-gases from such plants in many different locations. The underlying biochemical mechanisms are complex. The measured actual emission rates show high variability in N2O emissions, both in time and space (within a given bioreactor system) and between different bioreactors across a range of WWTP configurations. Under current GHG reporting protocols, both internationally and in Australia, fixed emission factors for N2O are typically used (i.e., invariant over time and source location). However, in the calculations underlying the emissions reported under different protocols, the way in which these factors are defined and applied can be different. Hence, aside from the complexity and variability of the actual biochemical mechanisms at play, emission factor definition and calculation methodology are a first potential source of confusion when reporting N2O emissions from WWTPs. A second source of potential confusion stems from the use of fixed emission factors (i.e., without reference to type of treatment plant or its performance, for example, in terms of nitrogen removal). A third source of confusion concerns the boundary of the WWTP and the distinction between N2O emissions from the WWTP treatment processes per se versus those associated with the discharge of WWTP effluent to a receiving water body (e.g., river, estuary ocean) or land (in the case of irrigation), and the disposal of biosolids.
This paper focusses on the first two potential sources of confusion around WWTP N2O emissions mentioned above. It starts with an overview, in simple terms, of the main biochemical mechanisms of N2O formation in activated sludge systems, based on current knowledge from research literature. Next, recent results (from both published literature and previously unpublished data, both overseas and in Australia) are examined, highlighting a common trend in average N2O emission factors from different WWTPs. Lastly, these results are compared with the N2O emission factors as defined in the IPCC (2019) and the current Australian NGER Determination (2020) reporting protocols.
Literature Review
N2O formation biochemical mechanisms in wastewater treatment
N2O formation in WWTPs have been previously reviewed by others (inter alia Law et al., 2012; Wunderlin et al., 2012, 2013; Valkova et al., 2021). In their review, Law et al. (2012) made following general observations:
- The microbial nitrogen transformation processes in a WWTP are fundamentally the same as in other environments such as soil, marine and freshwater 2 habitats. However, unlike most other environments, wastewater treatment plants are engineered systems designed to achieve high nitrogen conversion rates.
- Domestic wastewater usually contains relatively high concentrations of nitrogen (N), around 20-70 mg/L total nitrogen (TN) as N. To achieve almost complete nitrogen removal within short retention times (in the order of hours), high reactor N loading rates are applied, resulting in relatively high nitrification and denitrification rates, and potentially relatively high rates of N2O production.
- Bacterial communities in a WWTP are subjected to rapid changes in process conditions that are applied to promote aerobic or anoxic biochemical reactions. Such rapid changes likely cause physiological stress on both the nitrifying and denitrifying communities, and the potential to induce transient behaviours like N2O formation.
- Active aeration is used to induce aerobic conditions in WWTPs. The aeration systems are engineered to efficiently transfer oxygen to the bioreactor, but also enable efficient transfer of N2O from the liquid phase to the gas phase. Therefore, any temporary imbalance between N2O production and consumption in the bioreactor could result in N2O accumulation and/or stripping during aeration.
- Given that WWTPs are highly engineered, there are opportunities to mitigate N2O emissions by improving process design and/or operation.
The main pathways for N2O formation, based on the research literature of underlying biochemical mechanisms are summarised in Table 1. The two main pathways are autotrophic nitrification (via nitrifier denitrification; and/or hydroxylamine oxidation) and heterotrophic denitrification, with a third (chemical) pathway considered to be uncommon.

Sources: Goreau et al. (1980); Igarashi et al. (1997); Law et al. (2012); Ribera-Guardia et al. (2014); Soler-Jofra et al. (2016); Stein et al. (2011); Tallec et al. (2006); Valkova et al. (2021); von Schulthess et al. (1995); Wunderlin et al. (2013).
GHG reporting protocols
Two GHG reporting protocols for domestic wastewater treatment were reviewed, namely: the 2019 Refinement to the 2006 IPCC Guidelines for National Greenhouse Gas Inventories (international); and the National Greenhouse and Energy Reporting (Measurement) Determination 2008 (2020 compilation), which is applicable in Australia (Australian Govt, 2020).
In respect of N2O for domestic wastewater treated in centralised ‘aerobic’ treatment plants (not including effluent discharge), the IPCC guidelines (IPCC, 2019) apply a plant-wide fixed (default) emission factor (EFN2O-WWTP) of 0.016 kg N2O-N/kg N (1.6%) with respect to the influent (raw wastewater) total nitrogen (TN) load – N2O emissions are noted as ‘variable’ with a wide possible EF range given (0.016% – 4.5%) (IPCC, 2019). The IPCC default EFN2O-WWTP does not change with TN removal across the WWTP.
The origin of the IPCC (2019) default emission factor
(EFN2O-WWTP = 0.016 kg N2O-N/kg N) is presented in Figure1, found in Annex 6A.5 of the IPCC 2019 Refinement guidelines. The data for this chart was sourced from various literature references (cited by IPCC, 2019) for 30 WWTPs considered to be reflective of ‘the most typical and widely used treatment processes globally’ (IPCC, 2019). It is a reasonable supposition that the average N2O daily emission rate will be directly proportional to the TN mass loading rate (i.e., nominally the size of the WWTP), as suggested by the linear trend in Figure 1. However, the calculated slope of the trendline in Figure 1 (from which the IPCC default emission factor stems) was likely influenced heavily by the data from less than ten plants (a third of the dataset) with TN loading rates >4,000 kgN/d (approximately) i.e., relatively large WWTPs treating population equivalents of >300,000, indicatively. For many settings, including most of regional Australia and New Zealand, such large plants would be uncommon in terms of number. Moreover, the inherent assumption behind the trendline in Figure 1 is that process performance across the selected WWTPs, at least in terms of N removal, is essentially the same. In reality, N removal performance can differ widely between different WWTPs. The underlying biochemical mechanisms (see Table 1) suggest that N2O emissions are linked with N removal (i.e., nitrification-denitrification biological reactions) over a range of plant sizes from laboratory scale to full scale.

By contrast, for N2O from domestic wastewater treatment, the NGER Determination (Australian Govt., 2020) applies a plant-wide fixed EFN2O-WWTP of 2.082 tonnes CO2-e as N2O per tonne N “produced” (removed), which converts to 0.005 tonnes N2O-N per tonne N removed (0.5% of TN removed) at 265 tonnes CO2-e/ tonne N2O. For a case where treatment removal (by mass balance calculations in the NGER methodology) is indicatively 80% of influent TN, the NGER EF for N2O is equivalent to 0.4% of influent TN (i.e., four times lower than the IPCC default EF, see above). The NGER methodology provides no guidance on the range in the EF for N2O. However, when comparing the IPCC and NGER methods in equivalent terms (i.e., both relative to influent TN), the NGER EF effectively decreases with decreasing TN removal across the WWTP.
In both protocols (IPCC and NGER), the remainder of influent TN not removed (i.e., effluent TN discharged) is separately accounted for using a different EF for discharge defined in each of the respective guidelines, to account for N2O expected to be produced and released from the receiving environment. This aspect was not studied here.
Methodology
Actual N2O emissions data
We collated actual (measured) average N2O emissions and average TN removal data from a range of WWTPs, based on the recent literature data of Valkova et al. (2021) as provided by Parravicini (2020), for ten WWTPs in Europe, representing a total of twenty measurement campaigns in the period 2012-2018, including seasonal repetition.
We compared this dataset to that for eight WWTPs in Australia, representing eight measurement campaigns (1-2 months each, not seasonally repeated). Some of the Australian plant results have been published (Law et al., 2012; Ye et al., 2014; Pan et al., 2016; Duan et al., 2020), whilst the remainder (for three plants) are hitherto unpublished data collected by the University of Queensland. Trends in the datasets were investigated by regression analysis, and the results compared with emission factors applied in the IPCC (2019) and NGER (2020) reporting protocols.
Results and Discussion
Figure 2 shows that both the EU and AU datasets suggest linear trends in plant-wide EFN2O-WWTP where the EF is inversely related to TN removal (expressed in percentage terms) across the WWTP. The linear regression correlation is stronger for the EU dataset (R2 =0.86), which included more datapoints (n = 20), compared with the AU dataset (R2 =0.56, n= 7). One AU WWTP was excluded from the regression analysis since this measurement campaign was a special case (see Figure 2). The measured emissions were relatively low for this plant (considering its configuration and extent of TN removal, compared with other similar plants). Possible reasons for this were related to methodological differences around the off-gas measurement, and the presence of a thick scum/ foam layer on the surface of the aeration tanks in the vicinity of the gas hoods for off-gas collection. Biological removal of N2O through heterotrophic denitrification in the scum/ foam layer is possible.
The trendlines identified in Figure 2 imply that, in relative terms, N2O emissions increase as N removal decreases (i.e., where nitrification-denitrification reactions are incomplete or partial). Conversely, lower N2O emissions in relative terms, are expected to occur in WWTPs that achieve higher degrees of N removal (i.e., where nitrification-denitrification reactions are largely complete by the time the effluent leaves the bioreactors). Obviously, this trend has the inherent assumption that N removal occurs predominantly in one bioreactor (or one set of similar bioreactors). In practice, within the same WWTP, it is possible that different bioreactors (representing sequential or parallel process steps) emit N2O emissions to different degrees and remove N to different degrees, whereas the WWTP overall achieves a relatively high percentage of TN removal. In such cases, the WWTP data might give excursions from the trend in Figure 2.
As explained by Valkova et al. (2021), there are fundamental reasons why N2O emissions might be linked to the extent of TN removal across the mainstream processes for WWTPs. Essentially, most of the emitted N2O is attributed to autotrophic nitrification pathways. The biochemical mechanisms of N2O formation during nitrification are associated with higher ammonia oxidation rates, and/or higher reactor prevalence of partial nitrification/ denitrification products (e.g., nitrite), both of which are linked with higher reactor nitrogen loading rates, spatial feed distribution, DO concentrations, and transient aerobic conditions (e.g., at the start of aeration, moving from low to higher reactor DO). In each case, these conditions typically tend to occur in plants designed or operated for lower degrees of TN removal and/or shorter sludge ages. Plants that achieve near-complete TN removal tend to be designed for longer sludge ages and have larger reactors that are more lightly loaded, with larger anoxic fractions (N2O sinks).
Given the limitations from variance and number of datapoints in the datasets, Figure 2 suggests that the average EFN2O-WWTP, for a given degree of TN removal, is higher in the AU WWTPs (UQ dataset) than the EU WWTPs (Valkova et al., 2021 dataset). Many factors might contribute to variability in N2O emissions, for a given degree of TN removal (i.e., the vertical scatter of datapoints in Figure 2). Some of these might be physical factors around plant design and operation (e.g., aeration and recycles rates, reactor dissolved oxygen concentration, reactor type and configuration including compartmentalisation, feed and recycle set-up, flow and/or diurnal load patterns, reactor temperature etc.), while others might be biological factors (e.g., wastewater composition, microbial community diversity). Furthermore, given the difficulty of measuring off-gas or dissolved N2O in full scale reactors, methodological factors (systematic or measurement errors) likely also contribute to the EF uncertainty.
To compare reporting methodologies, the N2O EFWWTP can be expressed in two ways: (1) relative to influent TN load (e.g., IPCC, 2019); or (2) relative to TN removed (e.g., NGER, Australian Govt., 2020). Both approaches were examined for the datasets considered in this study.
N2O EF relative to Influent TN load
Figure 3 relates the actual N2O emissions data (from Figure 2) to the default emissions factors derived from the IPCC and NGER (equivalent) reporting protocols, expressed on a common basis, namely, N2O emissions with respect to percent influent TN load vs. percent TN removal across the whole WWTP. It shows that the IPCC EF (1.6%) is very conservative (i.e., high), makes no provision for the degree of TN removal and likely over-estimates actual N2O emissions for typical WWTPs. Conversely, the NGER EF (0.5% relative to TN removed in the 2020 compilation; decreased from 1.0% in previous NGER compilations) might only be representative of N2O emissions for WWTPs achieving at least approximately 80% TN removal. The formulation of the NGER EF as constant relative to TN removed results in the equivalent EF (relative to influent TN) decreasing with decreasing degrees of TN removal, whereas the actual N2O data suggests the opposite trend. The NGER methodology will therefore likely underestimate actual N2O emissions for WWTPs that achieve lower degrees of TN removal (indicatively <83% at EFN2O-WWTP = 0.4% in Figure 3).
Table 1 shows the outcome of correcting the EF’s applied in the IPCC and NGER methodologies, based on the ‘best fit’ linear regression for the pooled EU and AU datasets (excluding 1 no. AU WWTP, see above), as shown in Figure 4. The linear correlation for the pooled datasets is relatively weak (r2 = 0.55), as expected, given that the EU and AU datasets appear to be distinct from each other (Figure 2). Further work is required to understand the underlying reasons for such dataset differences, be they process related (e.g., temperature, type and configuration of bioreactors) or methodological (e.g., around N2O measurement campaigns, and/or TN removal calculation for the bioreactors per se or the WWTP as a whole).
N2O EF relative to TN removed
Since the predominant mechanisms for N2O formation in WWTPs involve biological pathways for nitrification and denitrification (Table 1), it is more sensible to relate the N2O emission factor to TN removal than influent TN.
Figure 5 presents the same datasets as for Figure 2, except with the emission factor (y-axis) expressed as a percentage relative to TN removed. Figure 5 shows improved linear correlations for both the EU and AU datasets, compared with Figure 2.
Figure 6 plots the actual N2O emissions data (from Figure 5) to the default emissions factors derived from the IPCC (equivalent) and NGER reporting protocols, expressed on the alternative common basis, namely, N2O emissions as a percentage of TN removed vs. percent TN removal across the whole WWTP. In this case, the IPCC equivalent emission factor (which is constant with respect to influent TN load, see Figure 3) increases as TN removal decreases (Figure 6) and at 100 % TN removal (theoretically) is numerically the same in percentage terms as the IPCC default (1.6% of influent TN load). The actual N2O data from both the EU and AU datasets reviewed here (Figure 6) suggest that the IPCC default is too conservative (too high).
The NGER default factor (constant with respect to TN removed, see Figure 6) might be more appropriate for WWTPs with TN removal in the higher range (indicatively 80 to 97%), but is likely to over-predict N2O emissions for some plants (e.g., most plants in the EU dataset in this range). Conversely, the data suggest that the NGER default factor is likely to under-predict N2O emissions in the lower range of TN removal (indicatively 60% to 80%), potentially by a large margin. The best approach would be to adopt a variable N2O emission factor, expressed with respect to percent TN removed (i.e., like the NGER approach) whereby the emission factor is a function of percent TN removal across the whole WWTP. Figure 7 shows a plot of the emission factor expressed on this basis for the pooled datasets (EU and AU) reviewed in this study. The correlation (r2 = 0.66) is somewhat better than that in Figure 4, but with room for improvement, potentially by adding more data from further field measurement campaigns (in Australia and internationally) and standardising measurement methodologies.
Finally, for context, 2015-16 TN removal data from a benchmarking study of WWTPs in Australia and New Zealand (de Haas et al., 2018) is summarised in Table 3. There is a wide variation in the range of actual TN removal for the WWTPs surveyed in this benchmarking study. Adopting the simple average of TN removal (83%) of all the WWTP surveyed (in 2015-16) might suggest that the NGER default factor is appropriate. However, using a weighted average of 56%TN removal (from Table 3 weighted on plant size i.e., EP, and hence mass of TN treated), by extrapolation from Figure 7, at national scale the NGER default factor is potentially under-estimating N2O emissions by a factor of more than four-fold. This could be corrected by adopting a variable emission factor for N2O reporting, based on TN removed for each plant, as suggested above.
Conclusion
Actual (measured) emissions data from the mainstream processes (typically activated sludge) of WWTPs in Europe and Australia suggest that N2O emissions (expressed relative to influent TN load), on average, increase as the degree of TN removal across the plant decreases. That is, less N2O is likely to be emitted as overall TN removal improves. The average N2O emission factor is likely to increase by at least 2 to 5-fold (and up to 10-fold or more) as the degree of TN removal decreases from >90% to <70%. As suggested recently by Valkova et al. (2021), linking the N2O emission factor to the degree of TN removal across a WWTP, is recommended as a better approach for GHG accounting than the use of fixed emission factors in current reporting protocols (e.g., IPCC and NGER). Additional full-scale measurement campaigns for N2O emissions from WWTPs, along with alignment of measurement and reporting methodologies, are needed to confirm the trends highlighted in this study. A review of the IPCC and NGER reporting methodologies for wastewater treatment N2O emissions is recommended in the light of these findings. In light of the identified trend toward N2O emissions with improved N removal, future work should include re-examination of the trade-offs for WWTPs between improved N removal (through more advanced treatment) and total GHG emissions. The total GHG footprint will take into account both direct emissions (including N2O and methane, potentially) and indirect emissions (i.e. grid electricity use and chemicals use, biosolids transport/ disposal etc), noting local or regional differences around indirect emission factors (e.g. the renewable energy component of grid electricity, and biosolids handling).



Note 1: Based on the “best fit” linear regression of pooled actual datasets in Figure 4.
Note 2: Based on the “best fit” linear regression of pooled actual datasets in Figure 7.

showing suggested corrected EF trendline from ‘best fit’ of pooled actual datasets, related on an equivalent basis of influent TN
load.

removed, versus percent of influent TN removal by the WWTP for European (EU) and Australian (AU) datasets. Note: One AU
WWTP (Special case) excluded from regression analysis.

with default values from IPCC or NGER protocols, related on an equivalent basis with respect to WWTP TN removed.

showing suggested corrected EF trendline for pooled actual datasets, related on an equivalent basis with respect to TN removed.

Note 1: Weighted average %TN removal contribution per Size Class (SC) to overall weighted average (ALL). Weighting from Adopted EP per WWTP relative to Total EP (for ALL plants).
EP: Equivalent population (persons) based on influent loading (COD or BOD and TKN)
Data sourced from de Haas et al. (2018)
About the authors
Dr David de Haas | Dr David de Haas is a Senior Technical Director at GHD Pty Ltd with thirty-five years’ experience in municipal water and wastewater treatment, covering research and development, planning, process design, operation, greenhouse gas emissions and energy assessments. He has worked in wastewater-related consultancy in Australia for more than twenty years.
Dr Liu Ye | Dr Liu Ye is an Associate Professor at the University of Queensland. She has more than twelve years’ experience in the field of fugitive GHG emissions from urban water/ wastewater systems. Her research outputs are used by Australian water utilities in full-scale treatment plants to reduce the fugitive GHG emissions
References
Australian Govt. (2020) National Greenhouse and Energy Reporting (Measurement) Determination 2008 made under subsection 10(3) of the National Greenhouse and Energy Reporting Act 2007- Compilation No. 12, 1 July 2020. Dept. of Industry, Science, Energy and Resources, Australian Government, Canberra.
de Haas, D.W., Appleby, G., Charakos, G. and Dinesh, N. (2018). Benchmarking energy use for wastewater treatment plants – A summary of the 2015-16 benchmarking study. Water e-Journal (Online journal of the Australian Water Association), vol. 3, no. 2, 2018. doi.org/10.21139/wej.2018.023.
Goreau, T., Kaplan, W., Wofsy, S., McElroy, M., Valois, F., Watson, S., 1980. Production of NO2- and N2O by nitrifying bacteria at reduced concentrations of oxygen. Appl. Environ. Microbiol. 40, 526–532.
IPCC (2019). Wastewater treatment and discharge. Bartram, D., Short, M.D., Ebie, Y., Farkaˇs, J., Gueguen, C., Peters, G.M., Zanzottera, N.M., Karthik, M., Masuda, S. In: Demirok, F.B., Herold, A. (Eds.), 2019 Refinement to the 2006 IPCC Guidelines for National Greenhouse Gas Inventories, Vol. 5 (Chapter 6).
Igarashi, N., Moriyama, H., Fujiwara, T., Fukumori, Y., Tanaka, N. (1997) The 2.8 A structure of hydroxylamine oxidoreductase from a nitrifying chemoautotrophic bacterium, Nitrosomonas europaea. Nat. Struct. Mol. Biol. 4, 276–284.
Law, Y., Ye, L., Pan, Y., Yuan, Z. (2012). Nitrous oxide emissions from wastewater treatment processes. Phil. Trans. R. Soc. B Biol. Sci. 367, 1265-1277.
Parravicini, V. (2020) Personal communication. TU Wien, Vienna, Austria. vparravi@iwag.tuwien.ac.at
Parravicini, V., Svardal, K., and Krampe, J. (2016) Greenhouse gas emissions from wastewater treatment plants. Energy Procedia 97 (2016), 246 – 253.
Ribera-Guardia, A., Pijuan, M., 2017. Distinctive NO and N2O emission patterns in ammonia oxidizing bacteria: effect of ammonia oxidation rate, DO and pH. Chem. Eng. J. 321, 358–365.
Soler-Jofra, A., Stevens, B., Hoekstra, M., Picioreanu, C., Sorokin, D., van Loosdrecht, M.C.M., P´erez, J., 2016. Importance of abiotic hydroxylamine conversion on nitrous oxide emissions during nitritation of reject water. Chem. Eng. J. 287, 720–726.
Stein, L.Y., 2011. Surveying N2O producing pathways in bacteria. Methods Enzymol. 486, 131–152.
Tallec, B., Billen, G., Garnier, G.A., Gousailles, M., 2006. Nitrous oxide emissions from secondary activated sludge in nitrifying conditions of urban wastewater treatment plants. Water Res. 40, 2972–2980.
Valkova, T., Parravicini, V., Saracevic, E., Tauber, J., Svardal, K., and Krampe, J. (2021) A method to estimate the direct nitrous oxide emissions of municipal wastewater treatment plants based on the degree of nitrogen removal. J. Environ. Manage. 279 (2021) 111563, 1-10.
von Schulthess, R., Kühni, M., Gujer, W., 1995. Release of nitric and nitrous oxides from denitrifying activated sludge. Water Res. 29, 215–226.
Wunderlin, P., Lehmann, M., Siegrist, H., Tuzson, B., Joss, A., Emmenegger, L., Mohn, J. (2013). Isotope signatures of N2O in a mixed microbial population system: constraints on N2O producing pathways in wastewater treatment. Environ. Sci. Technol. 47, 1339–1348.
Wunderlin, P., Mohn, J., Joss, A., Emmenegger, L., Siegrist, H. (2012). Mechanisms of N2O production in biological wastewater treatment under nitrifying and denitrifying conditions. Water Res. 46, 1027–1037.